
Advertisement

A Kid-friendly Introduction to Magnets and Magnetism
- Share Content on Facebook
- Share Content on LinkedIn
- Share Content on Flipboard
- Share Content on Reddit
- Share Content via Email
This explainer on magnets and magnetism is intended for our elementary and middle-school readers. If you're feeling magnetically drawn to read more on this topic, check out our long-form article How Magnets Work .
Magnets are fun to play with. It feels like you have a magic wand! Magnets are pieces of metal or rock with an invisible power to attract special kinds of metal. That power is called a force. In nature, a force is something that causes a push or a pull. Gravity is the force that keeps you from floating off the ground. Magnetism is the force that makes a magnet stick to your refrigerator.
The Earth Is a Magnet
Magnetism is at work all around you. Almost everything that uses electricity or runs with a motor has magnets in it. The magnetic force helps your car run, your microwave cook and your computer work. Even the Earth we live on is a giant magnet!
The middle of our planet is made of two metals called iron and nickel. Both metals are attracted to magnets. These special metals can also become magnets. Our planet is always turning around in space, and Earth's metal core is turning, too. These movements make a magnetic force that surrounds the Earth called a magnetic field.
A magnetic field is the area around a magnet that has magnetic force. All magnets have a magnetic field, no matter how big they are. Things that are attracted to magnets are called magnetic objects. All magnetic objects are made of metal, but not all metals are magnetic. The main metals that are attracted by magnets are iron, cobalt and nickel. You can turn one of these metals into a magnet by rubbing it with a magnet!
If a magnetic object like a paper clip enters a magnetic field, it is pulled toward the magnet. Magnetic fields even work under water. A strong magnet can even attract a magnetic object through a table.
Magnets Have Two Poles: North and South
A magnet has two ends, called poles. One end is the north pole, and the other is the south pole. Can you think of something else that has a north pole and a south pole? That's right — Earth is a lot like a magnet! Compasses work by magnetizing the rotating hand so it lines up with the magnetic poles of the planet.
If you hold two magnets in your hands, the north pole of one magnet will always attract the south pole of another. Opposite poles push each other away.
Because our planet is like a big magnet, it also has a magnetic field. Earth's magnetic field protects us from the sun's radiation. The North and South poles have natural colorful light shows called northern and southern lights. These lights are caused by sprays of energy coming off the sun bumping into the magnetic field of Earth.
The needle of a compass is a magnet, and it spins to line up with the poles of Earth, north and south.
Frequently Answered Questions
What is magnetism in magnets, what is the difference between magnet and magnetism.
Please copy/paste the following text to properly cite this HowStuffWorks.com article:
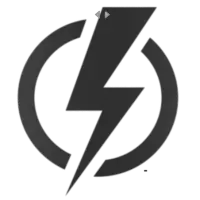
How does a magnetic paper work?
Explore the science behind magnetic paper, its composition, versatile applications, and how it revolutionizes education, organization, and decor.
Unraveling the Mystery of Magnetic Paper
Magnetic paper is a fascinating innovation that combines the properties of magnets with the flexibility and usability of paper. It has a myriad of applications, ranging from educational tools to creative projects and functional items. In this article, we will delve into the inner workings of magnetic paper and explore its various uses.
Understanding the Composition of Magnetic Paper
Magnetic paper is created by embedding small magnetic particles within a flexible, paper-like material. Typically, these particles consist of either iron or a ferrite-based compound. These particles are dispersed uniformly throughout the material, ensuring consistent magnetic properties across the entire surface.
The magnetic particles are then coated with a protective layer, usually made of plastic or a similar material, to prevent them from damaging the surrounding paper. This coating also makes the magnetic paper more durable and resistant to wear and tear.
Creating Custom Magnetic Surfaces
One of the primary advantages of magnetic paper is its ability to be easily cut and shaped into various forms, making it a versatile material for many applications. The most common method for cutting magnetic paper is using scissors or a cutting machine, which can create intricate shapes and patterns with ease.
Additionally, magnetic paper can be printed on using an inkjet or laser printer, allowing for the creation of custom designs and graphics. This feature makes magnetic paper an excellent choice for creating personalized items, such as fridge magnets, promotional materials, and educational tools.
Exploring the Many Uses of Magnetic Paper
- Educational Tools: Magnetic paper can be used to create interactive learning aids, such as magnetic maps, alphabet letters, and puzzle pieces. These tools can help students better understand and engage with various subjects, from geography to language arts.
- Organizational Aids: Magnetic paper is an ideal material for creating labels and signs that can be easily moved and rearranged on magnetic surfaces. This flexibility makes it perfect for use in offices, warehouses, and classrooms to keep track of inventory, schedules, and more.
- Decorative Items: With the ability to print custom designs and images on magnetic paper, it becomes an excellent medium for creating personalized and unique decorative items. These can include photo magnets, wall art, and other creative projects.
- Promotional Materials: Businesses and organizations can use magnetic paper to create custom promotional items, such as business cards, fridge magnets, and calendar magnets, which can be easily distributed and displayed on magnetic surfaces.
In conclusion, magnetic paper is a versatile and innovative material that combines the properties of magnets with the usability of paper. Its diverse applications and ease of customization make it an invaluable tool for educational, organizational, and creative purposes. As technology continues to advance, we can expect even more innovative uses for magnetic paper in the future.
Related Posts:
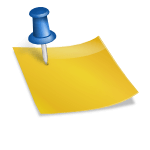
The primary purpose of this project is to help the public to learn some exciting and important information about electricity and magnetism.
Privacy Policy
Our Website follows all legal requirements to protect your privacy. Visit our Privacy Policy page.
The Cookies Statement is part of our Privacy Policy.
Editorial note
The information contained on this website is for general information purposes only. This website does not use any proprietary data. Visit our Editorial note.
Copyright Notice
It’s simple:
1) You may use almost everything for non-commercial and educational use.
2) You may not distribute or commercially exploit the content, especially on another website.

The Science of How Magnets Work
- Chemical Laws
- Periodic Table
- Projects & Experiments
- Scientific Method
- Biochemistry
- Physical Chemistry
- Medical Chemistry
- Chemistry In Everyday Life
- Famous Chemists
- Activities for Kids
- Abbreviations & Acronyms
- Weather & Climate
- Ph.D., Biomedical Sciences, University of Tennessee at Knoxville
- B.A., Physics and Mathematics, Hastings College
The force produced by a magnet is invisible and mystifying. Have you ever wondered how magnets work ?
Key Takeaways: How Magnets Work
- Magnetism is a physical phenomenon by which a substance is attracted or repelled by a magnetic field.
- The two sources of magnetism are electric current and spin magnetic moments of elementary particles (primarily electrons).
- A strong magnetic field is produced when the electron magnetic moments of a material are aligned. When they are disordered, the material is neither strongly attracted nor repelled by a magnetic field.
What Is a Magnet?
A magnet is any material capable of producing a magnetic field . Since any moving electric charge generates a magnetic field, electrons are tiny magnets. This electric current is one source of magnetism. However, the electrons in most materials are randomly oriented, so there is little or no net magnetic field. To put it simply, the electrons in a magnet tend to be oriented the same way. This happens naturally in many ions, atoms, and materials when they are cooled, but isn't as common at room temperature. Some elements (e.g., iron, cobalt, and nickel) are ferromagnetic (can be induced to become magnetized in a magnetic field) at room temperature. For these elements , the electrical potential is lowest when the magnetic moments of the valence electrons are aligned. Many other elements are diamagnetic . The unpaired atoms in diamagnetic materials generate a field that weakly repels a magnet. Some materials don't react with magnets at all.
The Magnetic Dipole and Magnetism
The atomic magnetic dipole is the source of magnetism. On the atomic level, magnetic dipoles mainly are the result of two types of movement of the electrons. There is the orbital motion of the electron around the nucleus, which produces an orbital dipole magnetic moment. The other component of the electron magnetic moment is due to the spin dipole magnetic moment. However, the movement of electrons around the nucleus isn't really an orbit, nor is the spin dipole magnetic moment associated with actual 'spinning' of the electrons. Unpaired electrons tend to contribute to a material's ability to become magnetic since the electron magnetic moment can't be totally canceled out when there are 'odd' electrons.
The Atomic Nucleus and Magnetism
The protons and neutrons in the nucleus also have orbital and spin angular momentum, and magnetic moments. The nuclear magnetic moment is much weaker than the electronic magnetic moment because although the angular momentum of the different particles may be comparable, the magnetic moment is inversely proportional to mass (mass of an electron is much less than that of a proton or neutron). The weaker nuclear magnetic moment is responsible for nuclear magnetic resonance (NMR), which is used for magnetic resonance imaging (MRI).
- Cheng, David K. (1992). Field and Wave Electromagnetics . Addison-Wesley Publishing Company, Inc. ISBN 978-0-201-12819-2.
- Du Trémolet de Lacheisserie, Étienne; Damien Gignoux; Michel Schlenker (2005). Magnetism: Fundamentals . Springer. ISBN 978-0-387-22967-6.
- Kronmüller, Helmut. (2007). Handbook of Magnetism and Advanced Magnetic Materials . John Wiley & Sons. ISBN 978-0-470-02217-7.
- What Is Magnetism? Definition, Examples, Facts
- Electron Definition: Chemistry Glossary
- Paramagnetism Definition and Examples
- Dipole Definition in Chemistry and Physics
- How to Tell If an Element Is Paramagnetic or Diamagnetic
- The Relationship Between Electricity and Magnetism
- Quantum Numbers and Electron Orbitals
- Learn What Metals Are Magnetic and Why
- Basic Model of the Atom and Atomic Theory
- Atoms and Atomic Theory - Study Guide
- Not All Iron Is Magnetic (Magnetic Elements)
- The Basics: An Introduction to Electricity and Electronics
- How to Demagnetize a Magnet
- FAQ: What is Electricity?
- Make an Atom Model
- Atom Definition and Examples
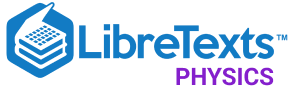
- school Campus Bookshelves
- menu_book Bookshelves
- perm_media Learning Objects
- login Login
- how_to_reg Request Instructor Account
- hub Instructor Commons
- Download Page (PDF)
- Download Full Book (PDF)
- Periodic Table
- Physics Constants
- Scientific Calculator
- Reference & Cite
- Tools expand_more
- Readability
selected template will load here
This action is not available.
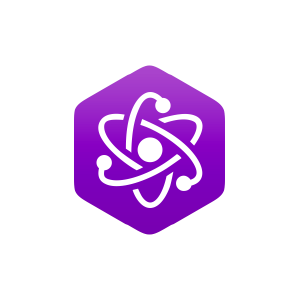
22.1: Magnets
- Last updated
- Save as PDF
- Page ID 2695
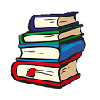
Learning Objectives
By the end of this section, you will be able to:
- Describe the difference between the north and south poles of a magnet.
- Describe how magnetic poles interact with each other.
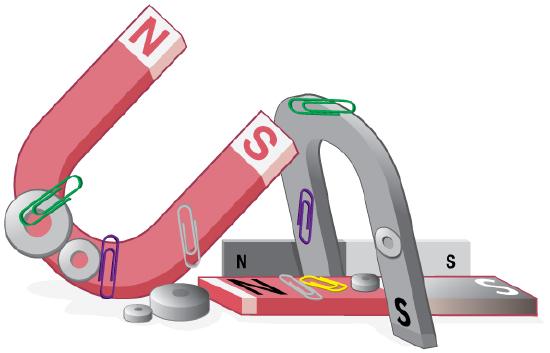
All magnets attract iron, such as that in a refrigerator door. However, magnets may attract or repel other magnets. Experimentation shows that all magnets have two poles. If freely suspended, one pole will point toward the north. The two poles are thus named the north magnetic pole and the south magnetic pole (or more properly, north-seeking and south-seeking poles, for the attractions in those directions).
UNIVERSAL CHARACTERISTICS OF MAGNETS AND MAGNET POLES
It is a universal characteristic of all magnets that like poles repel and unlike poles attract . (Note the similarity with electrostatics: unlike charges attract and like charges repel.)
Further experimentation shows that it is impossible to separate north and south poles in the manner that + and − charges can be separated.
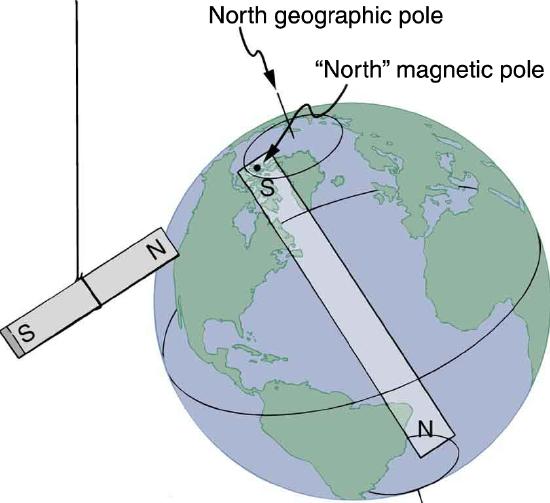
MISCONCEPTION ALERT: EARTH'S GEOGRAPHIC NORTH POLE HIDES AN S
The Earth acts like a very large bar magnet with its south-seeking pole near the geographic North Pole. That is why the north pole of your compass is attracted toward the geographic north pole of the Earth—because the magnetic pole that is near the geographic North Pole is actually a south magnetic pole! Confusion arises because the geographic term “North Pole” has come to be used (incorrectly) for the magnetic pole that is near the North Pole. Thus, “North magnetic pole” is actually a misnomer—it should be called the South magnetic pole.
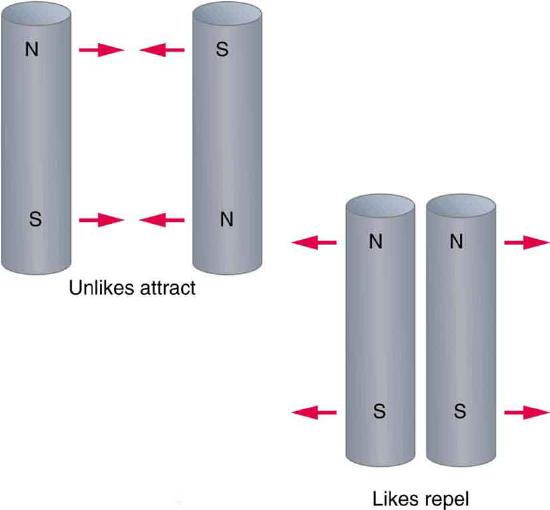
The fact that magnetic poles always occur in pairs of north and south is true from the very large scale—for example, sunspots always occur in pairs that are north and south magnetic poles—all the way down to the very small scale. Magnetic atoms have both a north pole and a south pole, as do many types of subatomic particles, such as electrons, protons, and neutrons.
MAKING CONNECTIONS: TAKE-HOME EXPERIMENT -- REFRIGERATOR MAGNETS
We know that like magnetic poles repel and unlike poles attract. See if you can show this for two refrigerator magnets. Will the magnets stick if you turn them over? Why do they stick to the door anyway? What can you say about the magnetic properties of the door next to the magnet? Do refrigerator magnets stick to metal or plastic spoons? Do they stick to all types of metal?
- Magnetism is a subject that includes the properties of magnets, the effect of the magnetic force on moving charges and currents, and the creation of magnetic fields by currents.
- There are two types of magnetic poles, called the north magnetic pole and south magnetic pole.
- North magnetic poles are those that are attracted toward the Earth’s geographic north pole.
- Like poles repel and unlike poles attract.
- Magnetic poles always occur in pairs of north and south—it is not possible to isolate north and south poles.
Physics Bootcamp
Samuel J. Ling
Section 38.2 Permanent and Temporary Magnets
Magnetism in magnets can be temporary or permanent. You are already familiar with permanent magnets, such as the refrigerator magnets. Permanent magnets are magnetized materials that have magnetism already built into them and whose magnetism is not easily destroyed.
Magnetic materials near a magnet also act as it they were magnets. For instance, a paper clip hanging from a magnet attracts other paper clips as illustrated on the right. The first paper clip is an example of a temporary magnet.
Similarly, electromagnets have magnetic property when current is flowing in the coil surrounding them. These are examples of Temporary magnets since their magnetism is not permanent.
Magnetizable materials, such as iron, cobalt, and nickel, can be transformed into permanent magnets by stroking the material with a permanent magnet as illustrated in Figure 38.2.2 .
First, place an unmagnetized iron bar on a table. Now, take a permanent magnet and rub the iron bar with the same pole of the magnet along the length of the iron bar in the same direction over and over again as indicated. The iron bar will become permanently magnetized!
Why do magnetizable materials become magnetized while other materials do not? You can trace the origin of magnetism in materials to the atomic level. Many atoms are tiny magnets themselves. In many materials, called ferromagnets or ferrimagnets , atomic magnets interact with each other such that they tend to align each other's poles.
In ferromagnets, the interatomic forces tend to align their magnetic poles, but thermal vibration tends to randomize them. If temperature is low enough, we find domains where atomic magnets are aligned overwhelmingly in one direction as shown in Figure 38.2.3 . When the domains are small and randomly oriented, the piece of material does not act as a permanent magnet, but when the domains are large and overall oriented in similar directions, the piece would be a permanent magnet.
When a material with atomic magnets is placed near a magnet, say near the South Pole of a permanent magnet, then the force of the external magnet tends to preferentially align the atomic magnets so that North Pole of the atomic magnet faces the South Pole of the external magnet. This leads to growth of magnetic domains that are aligned with the external magnet and large magnetic domains form that are oriented more or less in the direction of the external Poles.
Subsection 38.2.1 Electromagnets
In April of 1820 Hans Christian Øersted of University of Copenhagen (Denmark) published a remarkable discovery that united the subjects of electricity and magnetism. It is said that, while performing physics demonstrations for students, Øersted noticed that, whenever he turned on the current in the wire, a magnetic needle placed nearby also reacted to the current as illustrated in Figure 38.2.4 .
An electromagnet makes use of the magnetism of moving charges in current carrying wires. We will study this fundamental aspect of magnetism in the succeeding chapter. To enhance the impact of current, the wire carrying a current is wound in loops with current flowing in the same direction in each loop. The magnetic field of each loop of current add together to enhance their magnetic effect.
The magnetism of electromagnets can be demonstrated by bringing a permanent magnet near an electromagnet as shown in Figure 38.2.5 . The electromagnet acts just like a bar magnet with two poles since it attracts the North pole of the suspended permanent magnet when the current is one direction and repels the magnet when the orientation of the current is reversed. Electromagnet is an example of a temporary magnet that acts as a magnet when current through the material is on and as a non-magnet when the current is off.
Often, current carrying wires are wound around magnetizable material so that magnetic field of the current and the magnetized material add to produce a more powerful electromagnet. This is another way to transform a magnetizable material such as an iron bar into a permanent magnet. This is a preferred method of making new permanent magnets than by stroking the material.
Magnetic Fields
After reading this section you will be able to do the following:.
- Explain how magnetic lines of flux are affected by other magnetic fields.
In each of the following pictures a magnet is put onto a piece of paper. Then a light dusting of iron filings is sprinkled around the magnet. The lines around the magnets in the following pictures are produced by the iron filings gathering together around the field lines.
What does the pattern made by the iron particles indicate?
You learned in a previous experiment that no matter how many pieces you cut a magnet into, each piece is still a magnet. Even if you shred a magnet into particles the size of sand, each tiny grain is a magnet with a north pole and a south pole. When these magnetized particles are sprinkled over the magnet in Box A, the resulting pattern shows the magnetic field around a single magnet. We can see that the force of the magnet is the strongest at the two ends because more iron particles are concentrated in these areas. The magnetic lines of flux flow from one end to the other.
How do you explain what is occurring?
To understand what is happening, recall from a previous experiment that a magnet allowed to stand freely, like a compass needle, will point to the north in response to the earth’s magnetic field unless it is near a strong magnetic. If the compass is near a strong bar magnet, the opposite poles of the magnets are attracted to each other. We can use this knowledge to identify the magnetic field of a magnet by placing a compass at various locations around the bar magnet and observing where the compass needle points. If the compass is far away from the bar magnet the compass will always point north because it is not in the bar magnet’s magnetic field. As it gets closer to the magnet, the compass begins to point more and more toward the magnet as a result of the force, or the magnetic field, of the magnet. The compass needle aligns itself with the magnetic flux lines of the magnet.
Let's say that instead of using one compass to move around the bar magnet, we place thousands of tiny compass needles all around the bar magnet and watch which direction they point and what pattern they make. That is what is happening in our experiment with the iron filings. Each tiny magnetic iron filing is a tiny magnet with a north and south pole, just like a tiny compass. When the iron filings are sprinkled, those very close to the magnet, where the magnetic force is the strongest, will cling to the magnet.
Those filings a little farther away, where the magnetic force is less strong, will align themselves with the magnetic flux lines, but they will not be drawn to cling to the magnet. Those filings even farther away, outside the magnetic force, will point north in response to the earth’s magnetic field. These patterns formed by the direction of the tiny compasses can tell us something about where the magnetic force is the strongest, where it is an attracting force, and where it is a repelling force. In Box B, this pattern indicates a repelling force because the tiny magnets are moving away from the ends of the larger bar magnets. Looking at the pattern in Box C, you see that the two ends of these magnets are attracted because the tiny magnets appear to be lined end to end, attracting to one another and also attracting to the ends of the larger bar magnets.
- The force of the magnet is the strongest at the two ends.
20.1 Magnetic Fields, Field Lines, and Force
Section learning objectives.
By the end of this section, you will be able to do the following:
- Summarize properties of magnets and describe how some nonmagnetic materials can become magnetized
- Describe and interpret drawings of magnetic fields around permanent magnets and current-carrying wires
- Calculate the magnitude and direction of magnetic force in a magnetic field and the force on a current-carrying wire in a magnetic field
Teacher Support
The learning objectives in this section will help your students master the following standards:
- (G) investigate and describe the relationship between electric and magnetic fields in applications such as generators, motors, and transformers.
In addition, the High School Physics Laboratory Manual addresses content in this section in the lab titled: Magnetism, as well as the following standards:
Section Key Terms
Magnets and magnetization.
People have been aware of magnets and magnetism for thousands of years. The earliest records date back to ancient times, particularly in the region of Asia Minor called Magnesia—the name of this region is the source of words like magnet . Magnetic rocks found in Magnesia, which is now part of western Turkey, stimulated interest during ancient times. When humans first discovered magnetic rocks, they likely found that certain parts of these rocks attracted bits of iron or other magnetic rocks more strongly than other parts. These areas are called the poles of a magnet. A magnetic pole is the part of a magnet that exerts the strongest force on other magnets or magnetic material, such as iron. For example, the poles of the bar magnet shown in Figure 20.2 are where the paper clips are concentrated.
If a bar magnet is suspended so that it rotates freely, one pole of the magnet will always turn toward the north, with the opposite pole facing south. This discovery led to the compass, which is simply a small, elongated magnet mounted so that it can rotate freely. An example of a compass is shown Figure 20.3 . The pole of the magnet that orients northward is called the north pole , and the opposite pole of the magnet is called the south pole .
The discovery that one particular pole of a magnet orients northward, whereas the other pole orients southward allowed people to identify the north and south poles of any magnet. It was then noticed that the north poles of two different magnets repel each other, and likewise for the south poles. Conversely, the north pole of one magnet attracts the south pole of other magnets. This situation is analogous to that of electric charge, where like charges repel and unlike charges attract. In magnets, we simply replace charge with pole : Like poles repel and unlike poles attract. This is summarized in Figure 20.4 , which shows how the force between magnets depends on their relative orientation.
Consider again the fact that the pole of a magnet that orients northward is called the north pole of the magnet. If unlike poles attract, then the magnetic pole of Earth that is close to the geographic North Pole must be a magnetic south pole! Likewise, the magnetic pole of Earth that is close to the geographic South Pole must be a magnetic north pole. This situation is depicted in Figure 20.5 , in which Earth is represented as containing a giant internal bar magnet with its magnetic south pole at the geographic North Pole and vice versa. If we were to somehow suspend a giant bar magnet in space near Earth, then the north pole of the space magnet would be attracted to the south pole of Earth’s internal magnet. This is in essence what happens with a compass needle: Its magnetic north pole is attracted to the magnet south pole of Earth’s internal magnet.
What happens if you cut a bar magnet in half? Do you obtain one magnet with two south poles and one magnet with two north poles? The answer is no: Each half of the bar magnet has a north pole and a south pole. You can even continue cutting each piece of the bar magnet in half, and you will always obtain a new, smaller magnet with two opposite poles. As shown in Figure 20.6 , you can continue this process down to the atomic scale, and you will find that even the smallest particles that behave as magnets have two opposite poles. In fact, no experiment has ever found any object with a single magnetic pole, from the smallest subatomic particle such as electrons to the largest objects in the universe such as stars. Because magnets always have two poles, they are referred to as magnetic dipoles — di means two . Below, we will see that magnetic dipoles have properties that are analogous to electric dipoles.
Watch Physics
Introduction to magnetism.
This video provides an interesting introduction to magnetism and discusses, in particular, how electrons around their atoms contribute to the magnetic effects that we observe.
Grasp Check
Toward which magnetic pole of Earth is the north pole of a compass needle attracted?
- The north pole of a compass needle is attracted to the north magnetic pole of Earth, which is located near the geographic North Pole of Earth.
- The north pole of a compass needle is attracted to the south magnetic pole of Earth, which is located near the geographic North Pole of Earth.
- The north pole of a compass needle is attracted to the north magnetic pole of Earth, which is located near the geographic South Pole of Earth.
- The north pole of a compass needle is attracted to the south magnetic pole of Earth, which is located near the geographic South Pole of Earth.
Only certain materials, such as iron, cobalt, nickel, and gadolinium, exhibit strong magnetic effects. Such materials are called ferromagnetic , after the Latin word ferrum for iron. Other materials exhibit weak magnetic effects, which are detectable only with sensitive instruments. Not only do ferromagnetic materials respond strongly to magnets—the way iron is attracted to magnets—but they can also be magnetized themselves—that is, they can be induced to be magnetic or made into permanent magnets ( Figure 20.7 ). A permanent magnet is simply a material that retains its magnetic behavior for a long time, even when exposed to demagnetizing influences.
When a magnet is brought near a previously unmagnetized ferromagnetic material, it causes local magnetization of the material with unlike poles closest, as in the right side of Figure 20.7 . This causes an attractive force, which is why unmagnetized iron is attracted to a magnet.
What happens on a microscopic scale is illustrated in Figure 7(a) . Regions within the material called domains act like small bar magnets. Within domains, the magnetic poles of individual atoms are aligned. Each atom acts like a tiny bar magnet. Domains are small and randomly oriented in an unmagnetized ferromagnetic object. In response to an external magnetic field, the domains may grow to millimeter size, aligning themselves, as shown in Figure 7(b) . This induced magnetization can be made permanent if the material is heated and then cooled, or simply tapped in the presence of other magnets.
Conversely, a permanent magnet can be demagnetized by hard blows or by heating it in the absence of another magnet. Increased thermal motion at higher temperature can disrupt and randomize the orientation and size of the domains. There is a well-defined temperature for ferromagnetic materials, which is called the Curie temperature , above which they cannot be magnetized. The Curie temperature for iron is 1,043 K (770 °C °C ), which is well above room temperature. There are several elements and alloys that have Curie temperatures much lower than room temperature and are ferromagnetic only below those temperatures.
Refrigerator Magnets
We know that like magnetic poles repel and unlike poles attract. See if you can show this for two refrigerator magnets. Will the magnets stick if you turn them over? Why do they stick to the refrigerator door anyway? What can you say about the magnetic properties of the refrigerator door near the magnet? Do refrigerator magnets stick to metal or plastic spoons? Do they stick to all types of metal?
Holding a magnetic close to an unmagnetized ferromagnetic material will magnetically polarize the ferromagnetic material, causing the atomic magnetic dipoles to orient towards the external magnet. This is similar to electric polarization. Thus, the ferromagnetic material becomes magnetized in the presence of the external magnet, and the two magnets attract each other. For a magnet to stick to the refrigerator door, the door must contain some ferromagnetic material. Magnets will stick to ferrous spoons, for example spoons with iron in them, but not to nonferrous spoons, such as spoons made from Al or Ag, and will not stick to a magnet. Magnets will also not stick to plastic spoons.
You have one magnet with the north and south poles labeled. How can you use this magnet to identify the north and south poles of other magnets?
- If the north pole of a known magnet is repelled by a pole of an unknown magnet on bringing them closer, that pole of unknown magnet is its north pole; otherwise, it is its south pole.
- If the north pole of known magnet is attracted to a pole of an unknown magnet on bringing them closer, that pole of unknown magnet is its north pole; otherwise, it is its south pole.
Magnetic Fields
We have thus seen that forces can be applied between magnets and between magnets and ferromagnetic materials without any contact between the objects. This is reminiscent of electric forces, which also act over distances. Electric forces are described using the concept of the electric field, which is a force field around electric charges that describes the force on any other charge placed in the field. Likewise, a magnet creates a magnetic field around it that describes the force exerted on other magnets placed in the field. As with electric fields, the pictorial representation of magnetic field lines is very useful for visualizing the strength and direction of the magnetic field.
As shown in Figure 20.9 , the direction of magnetic field lines is defined to be the direction in which the north pole of a compass needle points. If you place a compass near the north pole of a magnet, the north pole of the compass needle will be repelled and point away from the magnet. Thus, the magnetic field lines point away from the north pole of a magnet and toward its south pole.
Magnetic field lines can be mapped out using a small compass. The compass is moved from point to point around a magnet, and at each point, a short line is drawn in the direction of the needle, as shown in Figure 20.10 . Joining the lines together then reveals the path of the magnetic field line. Another way to visualize magnetic field lines is to sprinkle iron filings around a magnet. The filings will orient themselves along the magnetic field lines, forming a pattern such as that shown on the right in Figure 20.10 .
Virtual Physics
Using a compass to map out the magnetic field.
This simulation presents you with a bar magnet and a small compass. Begin by dragging the compass around the bar magnet to see in which direction the magnetic field points. Note that the strength of the magnetic field is represented by the brightness of the magnetic field icons in the grid pattern around the magnet. Use the magnetic field meter to check the field strength at several points around the bar magnet. You can also flip the polarity of the magnet, or place Earth on the image to see how the compass orients itself.
With the slider at the top right of the simulation window, set the magnetic field strength to 100 percent . Now use the magnetic field meter to answer the following question: Near the magnet, where is the magnetic field strongest and where is it weakest? Don’t forget to check inside the bar magnet.
- The magnetic field is strongest at the center and weakest between the two poles just outside the bar magnet. The magnetic field lines are densest at the center and least dense between the two poles just outside the bar magnet.
- The magnetic field is strongest at the center and weakest between the two poles just outside the bar magnet. The magnetic field lines are least dense at the center and densest between the two poles just outside the bar magnet.
- The magnetic field is weakest at the center and strongest between the two poles just outside the bar magnet. The magnetic field lines are densest at the center and least dense between the two poles just outside the bar magnet.
- The magnetic field is weakest at the center and strongest between the two poles just outside the bar magnet and the magnetic field lines are least dense at the center and densest between the two poles just outside the bar magnet.
When two magnets are brought close together, the magnetic field lines are perturbed, just as happens for electric field lines when two electric charges are brought together. Bringing two north poles together—or two south poles—will cause a repulsion, and the magnetic field lines will bend away from each other. This is shown in Figure 20.11 , which shows the magnetic field lines created by the two closely separated north poles of a bar magnet. When opposite poles of two magnets are brought together, the magnetic field lines join together and become denser between the poles. This situation is shown in Figure 20.11 .
Like the electric field, the magnetic field is stronger where the lines are denser. Thus, between the two north poles in Figure 20.11 , the magnetic field is very weak because the density of the magnetic field is almost zero. A compass placed at that point would essentially spin freely if we ignore Earth’s magnetic field. Conversely, the magnetic field lines between the north and south poles in Figure 20.11 are very dense, indicating that the magnetic field is very strong in this region. A compass placed here would quickly align with the magnetic field and point toward the south pole on the right.
Misconception Alert
The density of the magnetic field lines in Figure 20.11 indicates the magnitude of the force that would be applied to a small test magnet placed in this field. The density does not indicate the force between the two magnets that create the field. The magnitude of the force between the two magnets is the same in both cases in Figure 20.11 . This can be understood by imagining that you place one of the magnets in the field of the other magnet. This situation is symmetrical: The magnetic fields look the same—other than direction—for both situations shown in Figure 20.11 . Because the magnets are of equal strength, they perturb the magnetic field of the opposite magnet, which is why the magnetic field must be probed by a small magnetic such as, a compass.
Note that magnets are not the only things that make magnetic fields. Early in the nineteenth century, people discovered that electrical currents cause magnetic effects. The first significant observation was by the Danish scientist Hans Christian Oersted (1777–1851), who found that a compass needle was deflected by a current-carrying wire. This was the first significant evidence that the movement of electric charges had any connection with magnets. An electromagnet is a device that uses electric current to make a magnetic field. These temporarily induced magnets are called electromagnets. Electromagnets are employed for everything from a wrecking yard crane that lifts scrapped cars to controlling the beam of a 90-km-circumference particle accelerator to the magnets in medical-imaging machines (see Figure 20.12 ).
The magnetic field created by an electric current in a long straight wire is shown in Figure 20.13 . The magnetic field lines form concentric circles around the wire. The direction of the magnetic field can be determined using the right-hand rule . This rule shows up in several places in the study of electricity and magnetism. Applied to a straight current-carrying wire, the right-hand rule says that, with your right thumb pointed in the direction of the current, the magnetic field will be in the direction in which your right fingers curl, as shown in Figure 20.13 . If the wire is very long compared to the distance r from the wire, the strength B of the magnetic field is given by
where I is the current in the wire in amperes. The SI unit for magnetic field is the tesla (T). The symbol μ 0 μ 0 —read “mu-zero”—is a constant called the “permeability of free space” and is given by
Magnetic Field Due to an Electric Current
This video describes the magnetic field created by a straight current-carrying wire. It goes over the right-hand rule to determine the direction of the magnetic field, and presents and discusses the formula for the strength of the magnetic field due to a straight current-carrying wire.
A long straight wire is placed on a table top and electric current flows through the wire from right to left. If you look at the wire end-on from the left end, does the magnetic field go clockwise or counterclockwise?
- By pointing your right-hand thumb in the direction opposite of current, the right-hand fingers will curl counterclockwise, so the magnetic field will be in the counterclockwise direction.
- By pointing your right-hand thumb in the direction opposite of current, the right-hand fingers will curl clockwise, so the magnetic field will be in the clockwise direction.
- By pointing your right-hand thumb in the direction of current, the right-hand fingers will curl counterclockwise, so the magnetic field will be in the counterclockwise direction.
- By pointing your right-hand thumb in the direction of current, the right-hand fingers will curl clockwise, so the magnetic field will be in the clockwise direction.
Now imagine winding a wire around a cylinder with the cylinder then removed. The result is a wire coil, as shown in Figure 20.14 . This is called a solenoid . To find the direction of the magnetic field produced by a solenoid, apply the right-hand rule to several points on the coil. You should be able to convince yourself that, inside the coil, the magnetic field points from left to right. In fact, another application of the right-hand rule is to curl your right-hand fingers around the coil in the direction in which the current flows. Your right thumb then points in the direction of the magnetic field inside the coil: left to right in this case.
Each loop of wire contributes to the magnetic field inside the solenoid. Because the magnetic field lines must form closed loops, the field lines close the loop outside the solenoid. The magnetic field lines are much denser inside the solenoid than outside the solenoid. The resulting magnetic field looks very much like that of a bar magnet, as shown in Figure 20.15 . The magnetic field strength deep inside a solenoid is
where N is the number of wire loops in the solenoid and ℓ ℓ is the length of the solenoid.
Electromagnets
Use this simulation to visualize the magnetic field made from a solenoid. Be sure to click on the tab that says Electromagnet. You can drive AC or DC current through the solenoid by choosing the appropriate current source. Use the field meter to measure the strength of the magnetic field and then change the number of loops in the solenoid to see how this affects the magnetic field strength.
- There will be no change in magnetic field strength when number of loops reduces from four to two.
- The magnetic field strength decreases to half of its initial value when number of loops reduces from four to two.
- The magnetic field strength increases to twice of its initial value when number of loops reduces from four to two.
- The magnetic field strength increases to four times of its initial value when number of loops reduces from four to two.
Magnetic Force
If a moving electric charge, that is electric current, produces a magnetic field that can exert a force on another magnet, then the reverse should be true by Newton’s third law. In other words, a charge moving through the magnetic field produced by another object should experience a force—and this is exactly what we find. As a concrete example, consider Figure 20.16 , which shows a charge q moving with velocity v → v → through a magnetic field B → B → between the poles of a permanent magnet. The magnitude F of the force experienced by this charge is
where θ θ is the angle between the velocity of the charge and the magnetic field. From this equation, we can see that the SI unit of magnetic field, the Tesla, is the magnetic field required to produce a 1 N force on a 1 C charge moving at 1 m/s in a direction perpendicular to the field and equivalent to the following: T = ( N ⋅ s ) ( C ⋅ m ) T = ( N ⋅ s ) ( C ⋅ m ) .
The direction of the force may be found by using another version of the right-hand rule: First, we join the tails of the velocity vector and a magnetic field vector, as shown in step 1 of Figure 20.16 . We then curl our right fingers from v → v → to B → B → , as indicated in step (2) of Figure 20.16 . The direction in which the right thumb points is the direction of the force. For the charge in Figure 20.16 , we find that the force is directed into the page.
Note that the factor sin θ sin θ in the equation F = q v B sin θ F = q v B sin θ means that zero force is applied on a charge that moves parallel to a magnetic field because θ = 0 θ = 0 and sin 0 = 0 sin 0 = 0 . The maximum force a charge can experience is when it moves perpendicular to the magnetic field, because θ = 90° θ = 90° and sin 90° = 1 . sin 90° = 1 .
Links To Physics
Magnetohydrodynamic drive.
In Tom Clancy’s Cold War novel “The Hunt for Red October,” the Soviet Union built a submarine (see Figure 20.17 ) with a magnetohydrodynamic drive that was so silent it could not be detected by surface ships. The only conceivable purpose to build such a submarine was to give the Soviet Union first-strike capability, because this submarine could sneak close to the coast of the United States and fire its ballistic missiles, destroying key military and government installations to prevent an American counterattack.
A magnetohydrodynamic drive is supposed to be silent because it has no moving parts. Instead, it uses the force experienced by charged particles that move in a magnetic field. The basic idea behind such a drive is depicted in Figure 20.18 . Salt water flows through a channel that runs from the front to the back of the submarine. A magnetic field is applied horizontally across the channel, and a voltage is applied across the electrodes on the top and bottom of the channel to force a downward electric current through the water. The charge carriers are the positive sodium ions and the negative chlorine ions of salt. Using the right-hand rule, the force on the charge carriers is found to be toward the rear of the vessel. The accelerated charges collide with water molecules and transfer their momentum, creating a jet of water that is propelled out the rear of the channel. By Newton’s third law, the vessel experiences a force of equal magnitude, but in the opposite direction.
Fortunately for all involved, it turns out that such a propulsion system is not very practical. Some back-of-the-envelope calculations show that, to power a submarine, either extraordinarily high magnetic fields or extraordinarily high electric currents would be required to obtain a reasonable thrust. In addition, prototypes of magnetohydrodynamic drives show that they are anything but silent. Electrolysis caused by running a current through salt water creates bubbles of hydrogen and oxygen, which makes this propulsion system quite noisy. The system also leaves a trail of chloride ions and metal chlorides that can easily be detected to locate the submarine. Finally, the chloride ions are extremely reactive and very quickly corrode metal parts, such as the electrode or the water channel itself. Thus, the Red October remains in the realm of fiction, but the physics involved is quite real.
- The current must flow vertically from up to down when viewed from the rear of the boat.
- The current must flow vertically from down to up when viewed from the rear of the boat.
- The current must flow horizontally from left to right when viewed from the rear of the boat.
- The current must flow horizontally from right to left when viewed from the rear of the boat.
Instead of a single charge moving through a magnetic field, consider now a steady current I moving through a straight wire. If we place this wire in a uniform magnetic field, as shown in Figure 20.19 , what is the force on the wire or, more precisely, on the electrons in the wire? An electric current involves charges that move. If the charges q move a distance ℓ ℓ in a time t , then their speed is v = ℓ / t . v = ℓ / t . Inserting this into the equation F = q v B sin θ F = q v B sin θ gives
The factor q / t in this equation is nothing more than the current in the wire. Thus, using I = q / t I = q / t , we obtain
This equation gives the force on a straight current-carrying wire of length ℓ ℓ in a magnetic field of strength B . The angle θ θ is the angle between the current vector and the magnetic field vector. Note that ℓ ℓ is the length of wire that is in the magnetic field and for which θ ≠ 0 , θ ≠ 0 , as shown in Figure 20.19 .
The direction of the force is determined in the same way as for a single charge. Curl your right fingers from the vector for I to the vector for B , and your right thumb will point in the direction of the force on the wire. For the wire shown in Figure 20.19 , the force is directed into the page.
Throughout this section, you may have noticed the symmetries between magnetic effects and electric effects. These effects all fall under the umbrella of electromagnetism , which is the study of electric and magnetic phenomena. We have seen that electric charges produce electric fields, and moving electric charges produce magnetic fields. A magnetic dipole produces a magnetic field, and, as we will see in the next section, moving magnetic dipoles produce an electric field. Thus, electricity and magnetism are two intimately related and symmetric phenomena.
Worked Example
Trajectory of electron in magnetic field.
A proton enters a region of constant magnetic field, as shown in Figure 20.20 . The magnetic field is coming out of the page. If the electron is moving at 3.0 × 10 6 m/s 3.0 × 10 6 m/s and the magnetic field strength is 2.0 T, what is the magnitude and direction of the force on the proton?
Use the equation F = q v B sin θ F = q v B sin θ to find the magnitude of the force on the proton. The angle between the magnetic field vectors and the velocity vector of the proton is 90° . 90° . The direction of the force may be found by using the right-hand rule.
The charge of the proton is q = 1.60 × 10 −19 C q = 1.60 × 10 −19 C . Entering this value and the given velocity and magnetic field strength into the equation F = q v B sin θ F = q v B sin θ gives
To find the direction of the force, first join the velocity vector end to end with the magnetic field vector, as shown in Figure 20.21 . Now place your right hand so that your fingers point in the direction of the velocity and curl them upward toward the magnetic field vector. The force is in the direction in which your thumb points. In this case, the force is downward in the plane of the paper in the z ^ z ^ -direction, as shown in Figure 20.21 .
Thus, combining the magnitude and the direction, we find that the force on the proton is ( 9.6 × 10 −13 N ) z ^ . ( 9.6 × 10 −13 N ) z ^ .
This seems like a very small force. However, the proton has a mass of 1.67 × 10 −27 kg 1.67 × 10 −27 kg , so its acceleration is a = F m = 9.6 × 10 −13 N 1.67 × 10 −27 kg = 5.7 × 10 14 m/s 2 a = F m = 9.6 × 10 −13 N 1.67 × 10 −27 kg = 5.7 × 10 14 m/s 2 , or about ten thousand billion times the acceleration due to gravity!
We found that the proton’s initial acceleration as it enters the magnetic field is downward in the plane of the page. Notice that, as the proton accelerates, its velocity remains perpendicular to the magnetic field, so the magnitude of the force does not change. In addition, because of the right-hand rule, the direction of the force remains perpendicular to the velocity. This force is nothing more than a centripetal force: It has a constant magnitude and is always perpendicular to the velocity. Thus, the magnitude of the velocity does not change, and the proton executes circular motion. The radius of this circle may be found by using the kinematics relationship.
The path of the proton in the magnetic field is shown in Figure 20.22 .
Wire with Current in Magnetic Field
Now suppose we run a wire through the uniform magnetic field from the previous example, as shown. If the wire carries a current of 1.5 A in the y ^ y ^ -direction, and the region with magnetic field is 4.0 cm long, what is the force on the wire?
Use equation F = I ℓ B sin θ F = I ℓ B sin θ to find the magnitude of the force on the wire. The length of the wire inside the magnetic field is 4.0 cm, and the angle between the current direction and the magnetic field direction is 90°. To find the direction of the force, use the right-hand rule as explained just after the equation F = I ℓ B sin θ . F = I ℓ B sin θ .
Insert the given values into equation F = I ℓ B sin θ F = I ℓ B sin θ to find the magnitude of the force
To find the direction of the force, begin by placing the current vector end to end with a vector for the magnetic field. The result is as shown in the figure in the previous Worked Example with v → v → replaced by I → I → . Curl your right-hand fingers from I → I → to B → B → and your right thumb points down the page, again as shown in the figure in the previous Worked Example. Thus, the direction of the force is in the x ^ x ^ -direction. The complete force is thus ( 0.12 N ) x ^ ( 0.12 N ) x ^ .
The direction of the force is the same as the initial direction of the force was in the previous example for a proton. However, because the current in a wire is confined to a wire, the direction in which the charges move does not change. Instead, the entire wire accelerates in the x ^ x ^ -direction. The force on a current-carrying wire in a magnetic field is the basis of all electrical motors, as we will see in the upcoming sections.
Practice Problems
What is the magnitude of the force on an electron moving at 1.0 × 10 6 m/s perpendicular to a 1.0-T magnetic field?
- 0.8 × 10 –13 N
- 1.6 × 10 –14 N
- 0.8 × 10 –14 N
- 1.6 × 10 –13 N
A straight 10 cm wire carries 0.40 A and is oriented perpendicular to a magnetic field. If the force on the wire is 0.022 N, what is the magnitude of the magnetic field?
- 1.10 × 10 –2 T
- 0.55 × 10 –2 T
Check Your Understanding
- Either the south pole of magnet 1 is closer to the north pole of magnet 2 or the north pole of magnet 1 is closer to the south pole of magnet 2.
- Either the south poles of both the magnet 1 and magnet 2 are closer to each other or the north poles of both the magnet 1 and magnet 2 are closer to each other.
- Not enough information is given to draw any conclusion about the orientation of the magnets.
Describe methods to demagnetize a ferromagnet.
- by cooling, heating, or submerging in water
- by heating, hammering, and spinning it in external magnetic field
- by hammering, heating, and rubbing with cloth
- by cooling, submerging in water, or rubbing with cloth
- The directional lines present inside and outside the magnetic material that indicate the magnitude and direction of the magnetic force.
- The directional lines present inside and outside the magnetic material that indicate the magnitude of the magnetic force.
- The directional lines present inside the magnetic material that indicate the magnitude and the direction of the magnetic force.
- The directional lines present outside the magnetic material that indicate the magnitude and the direction of the magnetic force.
As an Amazon Associate we earn from qualifying purchases.
This book may not be used in the training of large language models or otherwise be ingested into large language models or generative AI offerings without OpenStax's permission.
Want to cite, share, or modify this book? This book uses the Creative Commons Attribution License and you must attribute Texas Education Agency (TEA). The original material is available at: https://www.texasgateway.org/book/tea-physics . Changes were made to the original material, including updates to art, structure, and other content updates.
Access for free at https://openstax.org/books/physics/pages/1-introduction
- Authors: Paul Peter Urone, Roger Hinrichs
- Publisher/website: OpenStax
- Book title: Physics
- Publication date: Mar 26, 2020
- Location: Houston, Texas
- Book URL: https://openstax.org/books/physics/pages/1-introduction
- Section URL: https://openstax.org/books/physics/pages/20-1-magnetic-fields-field-lines-and-force
© Jan 19, 2024 Texas Education Agency (TEA). The OpenStax name, OpenStax logo, OpenStax book covers, OpenStax CNX name, and OpenStax CNX logo are not subject to the Creative Commons license and may not be reproduced without the prior and express written consent of Rice University.
What is Magnetization?
Experiment: Ask your mom or dad (or your teacher) for some metal paper-clips and a magnet. Can you pick up paper clips with the magnet? Can you pick up the paper clips with other paper clips?
Now, rub a paper clip with the magnet. Can you pick up other paper clips with it now?

ScienceSphere.blog
Magnetic Conversations: What Did The Paper Clip Say To The Magnet?

In this blog post, we will explore the concept of magnetic conversations through the perspective of two characters: the paper clip and the magnet. These inanimate objects may seem ordinary, but they have a lot to teach us about the power of communication and connection.
Table of Contents
Brief Explanation of Magnetic Conversations
Magnetic conversations refer to those interactions that draw people together, creating a strong bond and leaving a lasting impact. Just like how a magnet attracts a paper clip, these conversations have the ability to captivate our attention and create a sense of connection.
Introducing the Paper Clip and the Magnet
To better understand the concept of magnetic conversations, let’s introduce our characters: the paper clip and the magnet. The paper clip, a simple and unassuming object, represents our curiosity and desire to engage in conversation. On the other hand, the magnet symbolizes the captivating qualities that draw us towards certain individuals or topics.
As we delve into the perspectives of these characters, we will uncover valuable insights about the art of conversation and the importance of open-mindedness.
Stay tuned for the next section where we explore the paper clip’s perspective and its role in the magnetic conversation.
The Paper Clip’s Perspective
The paper clip, a simple yet versatile tool, plays a crucial role in our daily lives. It holds together important documents, keeps our papers organized, and serves as a symbol of efficiency. However, beyond its practical uses, the paper clip also has a unique perspective on the world around it.
Exploring the paper clip’s role and purpose
From the paper clip’s point of view, its purpose extends beyond its physical function. It sees itself as a connector, bringing together different pieces of information and ideas. It takes pride in its ability to keep things in order and create a sense of structure.
Discussing the paper clip’s curiosity about the magnet
Curiosity is a fundamental trait of the paper clip. It wonders about the magnet, a mysterious and powerful force that it often encounters. The paper clip is intrigued by the magnet’s ability to attract and repel objects, and it yearns to understand the science behind this phenomenon.
Highlighting the paper clip’s desire to engage in conversation
Despite being an inanimate object, the paper clip longs for connection and interaction. It desires to engage in conversations, not only with other paper clips but also with different objects it encounters. It believes that through conversation, it can gain new perspectives and expand its understanding of the world.
The paper clip’s perspective reminds us of the importance of curiosity and the desire to learn. It teaches us that even the simplest objects can have a unique outlook on life. By embracing curiosity, we can open ourselves up to new experiences and opportunities for growth.
In the next section, we will explore the magnet’s perspective and understand how these two characters come together in a magnetic conversation. Stay tuned for an intriguing insight into the magnet’s world.
The Magnet’s Perspective
The magnet, a powerful and enigmatic character in the conversation, holds a unique perspective on the interaction with the paper clip. Understanding the magnet’s characteristics and abilities is crucial to comprehending its attraction to the paper clip and its response to the paper clip’s curiosity.
Understanding the magnet’s characteristics and abilities
The magnet possesses an inherent magnetic field that exerts a force on objects within its range. This force, known as magnetism, is what draws the magnet to certain materials, including the paper clip. The magnet’s ability to attract and repel objects is a fundamental aspect of its nature.
The magnet’s magnetic field is created by the alignment of its atoms, which have tiny magnetic fields of their own. When these atoms align in the same direction, they create a stronger magnetic field. This alignment is achieved through a process called magnetization, which involves exposing the magnet to a strong external magnetic field or by rubbing it against another magnet.
Explaining the magnet’s attraction to the paper clip
The magnet’s attraction to the paper clip stems from the paper clip’s ferromagnetic properties. Ferromagnetic materials, such as iron or steel, contain atoms that can easily align with an external magnetic field. When the magnet comes into proximity with the paper clip, its magnetic field induces a magnetic response in the paper clip’s atoms, causing them to align with the magnet’s field.
This alignment creates a magnetic connection between the magnet and the paper clip, resulting in the paper clip being drawn towards the magnet. The strength of this attraction depends on various factors, including the distance between the magnet and the paper clip, the strength of the magnet’s magnetic field, and the magnetic properties of the paper clip.
Describing the magnet’s response to the paper clip’s curiosity
When the paper clip exhibits curiosity about the magnet, the magnet responds with a sense of intrigue and fascination. The magnet recognizes the paper clip’s willingness to engage in conversation and explore the mysteries of magnetism. It understands that the paper clip’s curiosity is a reflection of its own magnetic nature.
The magnet, being a silent observer, appreciates the opportunity to share its knowledge and experiences with the paper clip. It responds by revealing the wonders of magnetism, explaining the science behind its attraction and repulsion, and shedding light on the magnetic field it generates. The magnet’s response is filled with a sense of pride and satisfaction, knowing that it can contribute to the paper clip’s understanding of the world.
In this exchange, the magnet and the paper clip form a unique bond, a magnetic connection that transcends their physical properties. The magnet’s response to the paper clip’s curiosity deepens their connection, fostering a sense of mutual respect and admiration.
Understanding the magnet’s perspective is essential to grasp the intricacies of the conversation between the paper clip and the magnet. It allows us to appreciate the magnet’s role as a teacher and guide, imparting knowledge and wisdom to the curious paper clip. By delving into the magnet’s characteristics, attraction, and response, we gain a deeper understanding of the magnetic connection that binds these two characters together.
The Conversation Unfolds
In this section, we will delve into the dialogue between the paper clip and the magnet, showcasing the different perspectives and insights shared, and highlighting the magnetic connection between the two characters.
Presenting the dialogue between the paper clip and the magnet
The conversation between the paper clip and the magnet begins with the paper clip expressing its curiosity about the magnet. It asks the magnet, “What makes you so attractive? Why do I feel drawn to you?” The magnet, with a gentle hum, responds, “It’s my magnetic field. It’s a force that pulls objects like you towards me.”
The paper clip, intrigued by the magnet’s response, further inquires, “But why do you have this magnetic field? What purpose does it serve?” The magnet explains, “My magnetic field is a result of the alignment of my atoms. It allows me to attract certain materials, like iron or steel.”
Showcasing the different perspectives and insights shared
As the conversation progresses, the paper clip shares its perspective on its role and purpose. It reveals that its primary function is to hold papers together, keeping them organized and preventing them from getting lost. The magnet, listening attentively, acknowledges the importance of the paper clip’s role and appreciates its ability to bring order to chaotic situations.
The magnet then shares its own perspective, explaining that its purpose extends beyond attracting objects. It reveals that magnets are used in various applications, such as generating electricity, powering motors, and even in medical devices like MRI machines. The paper clip, amazed by the magnet’s versatility, realizes that there is much more to magnets than meets the eye.
Highlighting the magnetic connection between the two characters
Throughout the conversation, a magnetic connection between the paper clip and the magnet becomes evident. The paper clip, initially drawn to the magnet’s magnetic field, starts to understand that their connection goes beyond mere attraction. They both share a desire to engage in conversation, to learn from each other, and to explore the possibilities that arise from their interaction.
As the conversation unfolds, the paper clip and the magnet discover that their unique qualities and abilities complement each other. The paper clip’s organizational skills and the magnet’s magnetic field create a harmonious partnership, symbolizing the power of collaboration and synergy.
In this section, we witnessed the conversation between the paper clip and the magnet, where they shared their perspectives, insights, and formed a magnetic connection. This dialogue highlights the importance of communication and connection in our own lives.
By engaging in meaningful conversations, we can learn from others, gain new perspectives, and foster deeper connections. Just like the paper clip and the magnet, we can discover that our unique qualities and abilities can complement those of others, leading to powerful collaborations and positive outcomes.
So, let us embrace the concept of magnetic conversations, where curiosity, open-mindedness, and a willingness to engage can lead to transformative experiences. Let us seek out conversations that attract us, just like the paper clip was drawn to the magnet, and let us create connections that have the power to change our lives for the better.
Lessons Learned
In this section, we will delve into the significance of the conversation between the paper clip and the magnet and explore the valuable lessons we can learn from their interaction.
The Power of Communication and Connection
The conversation between the paper clip and the magnet highlights the importance of effective communication and meaningful connections. Communication is not just about exchanging words; it is about truly understanding and connecting with others. The paper clip’s curiosity and the magnet’s response demonstrate the power of engaging in conversations that go beyond surface-level interactions.
When we engage in meaningful conversations , we open ourselves up to new perspectives, ideas, and insights. These conversations allow us to broaden our horizons and gain a deeper understanding of the world around us. By actively listening and engaging in dialogue, we can foster stronger connections with others and build more meaningful relationships.
The Role of Open-Mindedness and Curiosity
The paper clip’s curiosity about the magnet serves as a reminder of the importance of open-mindedness . By being open to new experiences and ideas, we can expand our knowledge and challenge our preconceived notions. The paper clip’s willingness to engage in conversation with the magnet, despite their differences, demonstrates the power of embracing diversity and seeking out different perspectives.
Curiosity is a catalyst for growth and learning. It fuels our desire to explore, question, and seek answers. The paper clip’s curiosity about the magnet not only leads to a fascinating conversation but also encourages us to embrace our own curiosity. By asking questions and seeking knowledge, we can continuously learn and grow.
Embracing Differences and Finding Common Ground
The conversation between the paper clip and the magnet showcases the beauty of embracing differences and finding common ground. Despite their contrasting characteristics, the paper clip and the magnet are able to connect and engage in a meaningful conversation.
In our own lives, we encounter people with different backgrounds, beliefs, and perspectives. Instead of focusing on our differences, we can strive to find common ground and build bridges of understanding. By recognizing and appreciating the unique qualities and strengths of others, we can foster a sense of unity and create a more inclusive and harmonious world.
The conversation between the paper clip and the magnet serves as a metaphor for the power of magnetic conversations in our own lives. Through effective communication, open-mindedness, and embracing differences, we can create meaningful connections and gain valuable insights.
As we reflect on the lessons learned from the paper clip and the magnet, let us remember the importance of engaging in conversations that go beyond the surface. Let us embrace curiosity, open-mindedness, and a willingness to connect with others. By doing so, we can foster deeper relationships, broaden our perspectives, and create a more interconnected world. So, let’s start having more magnetic conversations and see the transformative impact they can have on our lives.
Decoding Racial Triangulation: Unveiling Its Impact On English Language
Decoding The P Vs. Q Inventory Systems: Unveiling The Key Differences
Unveiling The Mystery: What Is Milk Whitener And How Does It Work?
Decoding The Mystery: What Exactly Is A Dna Size Standard?
Unveiling The Secrets: What Is Hearth Bread And Why It’s A Must-Try
Decoding The Mystery: What Time Is 22 32 In English Language?
Decoding Labor Demand: Unveiling The Elasticity Mystery
Demystifying Abrams Law: Understanding Its Impact In English Language
Curiosity Bites: What Happens If You Eat Concrete?
Decoding The Sexual Slang: What Does “Hh” Mean?
Leave a Comment Cancel reply
Save my name, email, and website in this browser for the next time I comment.
Item added to your cart
Everything you need to know about using inkjet printable magnetic paper.
Everything You Need to Know About Using Inkjet Magnetic Paper
Inkjet magnetic paper has become increasingly popular in recent years as a versatile and creative option for printing various materials. Whether you're looking to make personalized magnets for your fridge or design eye-catching marketing materials, inkjet magnetic paper offers endless possibilities. In this article, we will explore everything you need to know about using inkjet magnetic paper and how to unleash your creativity.
What is Inkjet Magnetic Paper?
Inkjet magnetic paper is a specially designed material that combines the convenience of printable paper with the magnetic properties of magnets. It allows you to print your designs, artwork, or text using an inkjet printer and then turn them into magnets or other magnetic materials. The printable side of the paper is coated to receive ink from an inkjet printer, while the other side features a magnetic backing that allows the paper to adhere to metal surfaces.
How Does Inkjet Magnetic Paper Work?
Using inkjet magnetic paper is straightforward. Once you have your design ready, you can load the inkjet magnetic paper into your inkjet printer, making sure to insert it correctly based on the printer's guidelines. It's essential to choose the appropriate print settings for the best results. Generally, selecting the photo or high-quality printing option will provide vibrant colors and sharp details.
After printing, allow the ink to dry completely before handling the paper. Once dry, you can cut out the printed designs using scissors, a craft knife, or a paper cutter, depending on your preference and the complexity of the shapes. Peel off the protective backing to reveal the adhesive magnetic side, and your printed designs are ready to stick to any magnetic surface.
What Can You Create with Inkjet Magnetic Paper?
The possibilities for using inkjet magnetic paper are practically limitless. Here are some exciting ideas to inspire your creativity:
Custom Magnets: Personalize your fridge or magnetic board by printing photos, quotes, or artwork onto inkjet magnetic paper. It's a fantastic way to showcase your favorite memories or add a touch of uniqueness to your space.
Promotional Materials: Create eye-catching promotional magnets for your business. Print your logo, contact information, or special offers on inkjet magnetic paper and distribute them to potential customers. They can serve as memorable and useful reminders of your brand.
Educational Tools: Design educational magnets for children by printing letters, numbers, shapes, or interactive learning materials. These magnets can make learning fun and engaging for kids.
Party Favors and Gifts: Craft personalized party favors or unique gifts using inkjet magnetic paper. Print custom designs or messages to create memorable keepsakes for birthdays, weddings, or other special occasions.
Organizational Tools: Print labels or signs on inkjet magnetic paper to help you stay organized. Use them on metal storage bins, filing cabinets, or whiteboards for easy repositioning.
Tips for Using Inkjet Magnetic Paper:
To ensure the best results when using inkjet magnetic paper, consider the following tips:
Choose the Right Printer: Inkjet magnetic paper works well with most inkjet printers, but it's essential to check your printer's specifications to ensure compatibility.
Test Print: Before printing your final designs, consider doing a test print on regular paper to verify colors, layout, and alignment. This way, you can make any necessary adjustments before using the inkjet magnetic paper.
Handle with Care: Inkjet magnetic paper is relatively thin and can be delicate. Handle it carefully to avoid tearing or creasing the paper, which could affect the final results.
Store Properly: Keep your inkjet magnetic paper in a cool, dry place, away from direct sunlight or moisture, to maintain its quality and adhesive properties.
Experiment and Have Fun: Inkjet magnetic paper offers a great opportunity to experiment with different designs, colors, and shapes. Let your creativity run wild and have fun exploring all the possibilities.
Inkjet magnetic paper is a fantastic tool for adding a personal touch to your surroundings, promoting your business, or creating unique gifts. With a little imagination and the right printing techniques, you can turn ordinary prints into extraordinary magnets. So grab some inkjet magnetic paper and let your creativity soar!
- Choosing a selection results in a full page refresh.
- Opens in a new window.
- The Woodlands

Just in time for this year's Charles Schwab Challenge, Fort Worth's Colonial Golf Course has undergone a makeover.
A fabled red plaid jacket awaits the winner of the 2024 Charles Schwab Challenge. (Photo by Erich Schlegel-USA TODAY Sports)
2023 winner Emiliano Grillo sips champagne seated next to the Leonard Trophy.
The Wall of Champions tells the storied history of the longest-running PGA Tournament.
Fort Worth’s Colonial Golf Course Gets A Major Facelift Before The Annual Charles Schwab Challenge
New state-of-the-art improvements, same time-honored traditions.

A lot has changed at Fort Worth’s Colonial Country Club since it hosted the 2023 Charles Schwab Challenge. The storied course that Marvin Leonard opened in 1936, and then introduced in legendary fashion as it hosted the 1941 U.S. Open, has achieved a major make-over ― modernizing and renovating the oak-lined fairways to great effect.
Renowned golf course architects Gil Hanse (who also unveiled PGA Frisco in 2022) and Jim Wagner embarked on the most significant renovation project in the course’s storied history. This year’s tournament will serve as its big reveal.
The project addressed every aspect of the course infrastructure, including a state-of-the-art irrigation system as well as new bunkers, tees, and greens ― so while PGA Tour pros who have played Colonial many times, return to Fort Worth this May, it won’t be the same course they remember.
“It will still be the revered Colonial course we are all familiar with seeing and playing, but the Hanse work will improve, update, and maximize this legendary course and routing,” says Colonial Country Club GM Frank Cordeiro of the major overhaul.
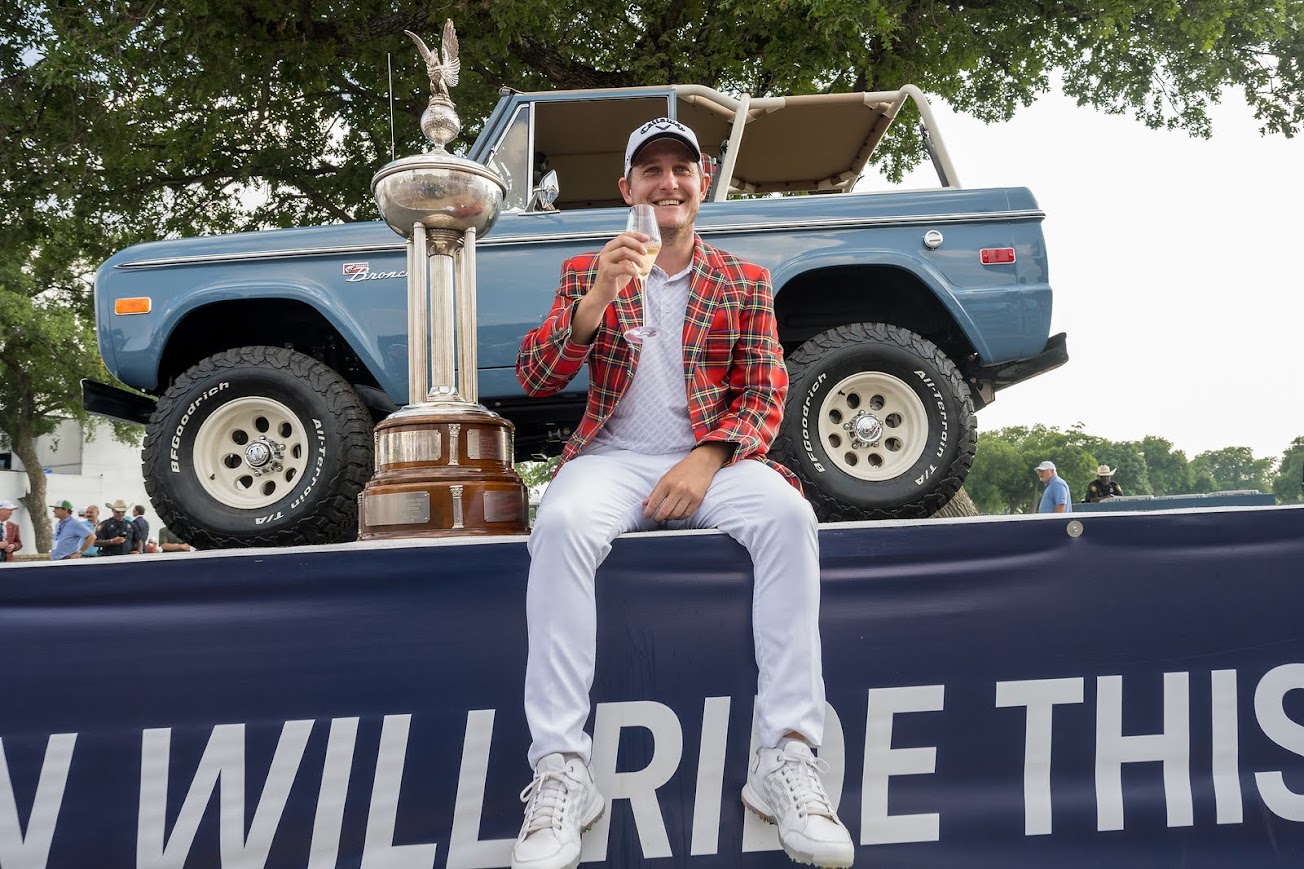
Historic Re-Envisioning
State-of-the-art improvements like new subterranean cooling and heating systems for the green complexes will manage and adjust soil temperatures for superior growth and plant health ― maintaining the legendary landscape throughout North Texas’ notoriously wild weather swings.
The biggest changes to the course were on par three holes No. 8 and No. 13, which were drastically changed by the 1969 flood-control project, which was undertaken by the Corps of Engineers ― dramatically altering the Trinity River channel, and forming the north border of the golf course .
Discover the Sweetness of Peche: Shop Le Creuset's Newest Color at Bering's!
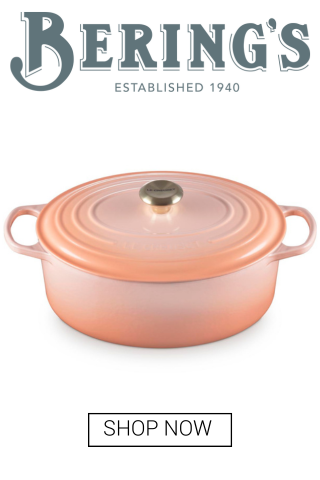
“The par three eighth hole was shifted left into more of a north-to-south orientation, in somewhat of a mirror image of the original hole, with a creek on the left side rather than a river on the right,” according to a release. “The 13th green also moved back and left, with bunkers added in front of the hole.”
Founder Marvin Leonard famously brought bentgrass greens to Texas for the first time against all odds, along with his course architects John Bredemus and Perry Maxwell. In fact, Leonard founded Colonial first as his own private club ― to see his vision of seeding superior bentgrass come to life. Once his gamble proved a success, he utilized the same grass strain at the next country club he founded ― Shady Oaks, as well as on his family ranch in Tolar, with its own nine-hole wonder ― Starr Hollow .
But, after withstanding 87 brutal Texas summers, the grass will now be nurtured from underneath ― by a hydronic water system that tricks the grass into thinking it has prime weather conditions all year long. Colonial will surely be the envy of other North Texas golf clubs for many years to come.
Most greens have now been lowered, and some have shifted slightly back or to either side a few yards. And, fans and golfers alike will notice “barrancas” incorporated throughout the course. A barranca is a normally dry streambed that channels water during occurrences of heavy rain. The new barrancas will be seen on holes 5, 6, 7, 8, 10, 15, 16, 17, and 18 ― making the most of the existing, natural drainage channels on the property.
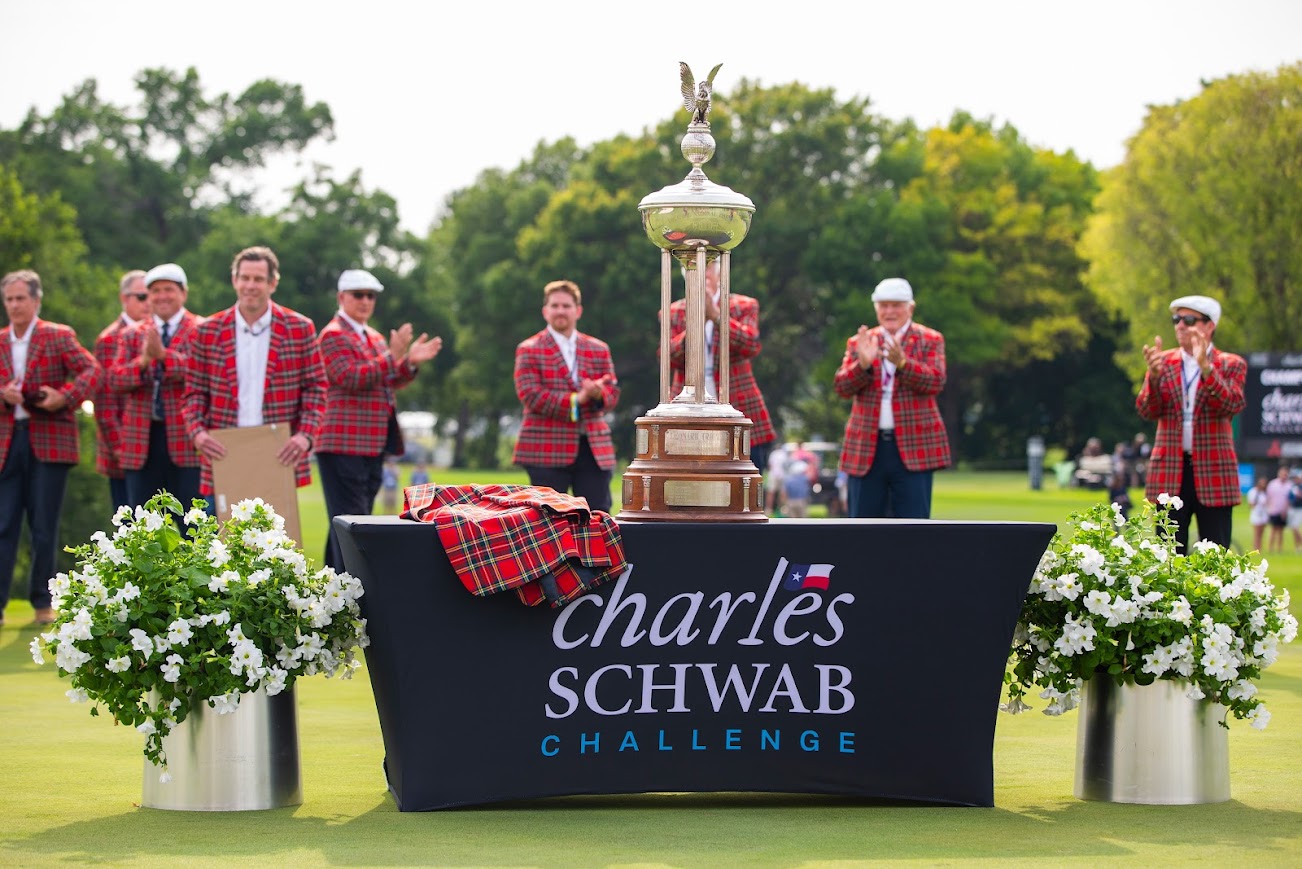
Time-Tested Traditions
Some things at Colonial never change. At 78 years and counting, Colonial boasts by far the longest-running PGA Tour-sponsored event held at the same golf course, and this year the course is looking younger and fresher than ever, following its profound nip and tuck.
The annual pilgrimage will bring the best golfers in the world to town between May 20 and May 26. They’ll, no doubt be awed by the course’s major transformation. But, they’re here on a business trip after all ― each vying to win their portion of the Charles Schwab Challenge’s massive purse.
No matter how you “slice” it ― $9,100,000 is an awful big pie.
These PGA pros will still have to make their way through Hogan’s Alley, named after Ben Hogan who won the tournament here five times ― holding practically every record on the Colonial Country Club course at one time or another. Then, the 2024 winner will be draped in a red plaid jacket, hoist the Leonard Trophy, and have his name forever etched into the Wall of Champions.
Tickets for the Charles Schwab Challenge, ranging from The Villages, The Patio Club, and General Admission are on sale now .
ReuNight for The Family Place
- 6:00 - 10:00 pm
Make-A-Wish North Texas Wish Night Gala
- 6:00 - 11:00 pm
Mother’s Day Brunch at Sorriso
- 10:00 - 3:00 pm
Mother’s Day Brunch
- 10:00 - 2:00 pm
2024 LBJ Moral Courage Award Dinner
- 6:00 - 9:00 pm
Parker McCollum and Friends: ACM Lifting Lives LIVE
- 7:45 - 10:00 pm
Ivo Morrison | The Introduction
Covenant house texas “night of broadway stars”, 59th academy of country music awards.
- 7:00 - 10:00 pm
Beauty and the Beast Fort Worth opening night dinner
- 5:30 - 10:00 pm
Methodist Health System Foundation’s Robert S. Folsom Leadership Award
- 6:30 - 9:00 pm

Luck of the Draw
Tom hoitsma open studio.
- 4:00 - 7:00 pm

Featured Properties
_md.jpg)
1025 Crocker Street Houston, TX
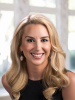
25 Beacon Hill Sugar Land, TX
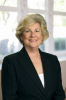
4206 Silver Reef Court Galveston, TX
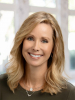
14 Greenway Plaza #30F Houston, TX
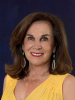
Like PaperCity Dallas on Facebook
Beyond the magazine. Get more of Dallas’ top restaurant, real estate, society, fashion and art in your news feed.
Create a free account to save your favorite PaperCity content in one curated collection.
Email Address *
Password *
Create Account
Already have an account? Login
Login to your account
Remember me
Lost your password?
Don't have an account? Create Account
Create a free account to view all PaperCity recipes. Save all of your favorite content in one curated collection.
Get PC Daily delivered directly to your inbox – don't miss anything!
Search PaperCityMag.com
Help | Advanced Search
Condensed Matter > Strongly Correlated Electrons
Title: simulating spin dynamics of supersolid states in a quantum ising magnet.
Abstract: Motivated by the recent experimental study on a quantum Ising magnet $\text{K}_2\text{Co}(\text{SeO}_3)_2$ where spectroscopic evidence of zero-field supersolidity is presented [arXiv: 2402.15869 ], we simulate the excitation spectrum of the corresponding microscopic $XXZ$ model for the compound, using the recently developed excitation ansatz of infinite projected entangled-pair states (iPEPS). We map out the ground state phase diagram and compute the dynamical spin structure factors across a range of magnetic field strengths, focusing especially on the two supersolid phases found near zero and saturation fields. Our simulated excitation spectra for the zero-field supersolid "Y" phase are in excellent agreement with the experimental data -- recovering the low-energy branches and integer quantized excited energy levels $\omega_n=nJ_{zz}$. Furthermore, we demonstrate the nonlocal multi-spin-flip features for modes at $\omega_2$, indicative of their multi-magnon nature. Additionally, we identify characteristics of the high-field supersolid "V" phase in the simulated spectra, to be compared with future experimental results.
Submission history
Access paper:.
- Other Formats

References & Citations
- Google Scholar
- Semantic Scholar
BibTeX formatted citation

Bibliographic and Citation Tools
Code, data and media associated with this article, recommenders and search tools.
- Institution
arXivLabs: experimental projects with community collaborators
arXivLabs is a framework that allows collaborators to develop and share new arXiv features directly on our website.
Both individuals and organizations that work with arXivLabs have embraced and accepted our values of openness, community, excellence, and user data privacy. arXiv is committed to these values and only works with partners that adhere to them.
Have an idea for a project that will add value for arXiv's community? Learn more about arXivLabs .
share this!
May 2, 2024
This article has been reviewed according to Science X's editorial process and policies . Editors have highlighted the following attributes while ensuring the content's credibility:
fact-checked
peer-reviewed publication
trusted source
Weak magnetic field may have supported diversification of life on Earth
by Nature Publishing Group

An unusual reduction in the strength of Earth's magnetic field between 591 and 565 million years ago coincided with a significant increase in the oxygen levels in the atmosphere and oceans, according to a paper published in Communications Earth & Environment . The authors propose that the weakening of the magnetic field may have led to the increase in oxygen, which is believed to have supported the evolution of some of the earliest complex organisms.
Between 600 and 540 million years ago, life on Earth consisted of soft-bodied organisms known as the Ediacaran fauna, the earliest known complex multicellular animals. The fossil record shows that these organisms significantly diversified in complexity and type between 575 and 565 million years ago. Previous research has suggested that this diversification is linked to a significant increase in atmospheric and oceanic oxygen levels that occurred over the same period. However, it is not yet clear why this increase in oxygen occurred.
Researcher John Tarduno and colleagues analyzed the magnetic properties of 21 plagioclase crystals, a common mineral in Earth's crust, which were extracted from a 591-million-year-old rock formation in Brazil. Plagioclase crystals contain tiny magnetic minerals that preserve the intensity of the Earth's magnetic field at the time they are formed.
Analysis of the crystals showed that at their point of formation, Earth's magnetic field was the weakest ever recorded—some 30 times weaker than both the current magnetic field intensity, and that measured from similar crystals formed approximately 2,000 million years ago.
The authors combined their results with previous measurements to establish that the Earth's magnetic field was at this weak level for at least 26 million years, from 591 to 565 million years ago. This overlaps with the rise in oxygen, which occurred between 575 and 565 million years ago.
The authors propose that the weakened magnetic field may have allowed more hydrogen to escape to space, resulting in a greater percentage of oxygen in Earth's atmosphere and oceans, which may in turn have supported the diversification in the types and complexity of organisms.
Journal information: Communications Earth & Environment
Provided by Nature Publishing Group
Explore further
Feedback to editors

New phononics materials may lead to smaller, more powerful wireless devices
4 hours ago

New 'forever chemical' cleanup strategy discovered
5 hours ago

TESS discovers a rocky planet that glows with molten lava as it's squeezed by its neighbors

Ultrasound experiment identifies new superconductor
6 hours ago

Quantum breakthrough sheds light on perplexing high-temperature superconductors

How climate change will affect malaria transmission
7 hours ago

Topological phonons: Where vibrations find their twist

Looking for life on Enceladus: What questions should we ask?
8 hours ago

Analysis of millions of posts shows that users seek out echo chambers on social media

NASA images help explain eating habits of massive black hole
Relevant physicsforums posts, the secrets of prof. verschure's rosetta stones, a very puzzling rock or a pallasite / mesmosiderite or a nothing burger.
23 hours ago
M 4.8 - Whitehouse Station, New Jersey, US
May 8, 2024
What is global warming due to?
Large eruption at ruang volcano, indonesia, tidal friction and global warming.
Apr 20, 2024
More from Earth Sciences
Related Stories

Researchers find oldest undisputed evidence of Earth's magnetic field
Apr 24, 2024

Ancient crystals offer evidence of the start of Earth's core solidifying
Jan 29, 2019

Cosmic rays streamed through Earth's atmosphere 41,000 years ago: New findings on the Laschamps excursion
Apr 19, 2024

How did Earth avoid a Mars-like fate? Ancient rocks hold clues
Jul 25, 2022

New research provides evidence of strong early magnetic field around Earth
Jan 20, 2020

Antarctic lava yields clues to Earth's past magnetic field
Feb 4, 2021
Recommended for you

Improved wildfire smoke model identifies areas for public health intervention

Unearthing the impacts of hydrological sensitivity on global rainfall
12 hours ago

Computer models suggest modern plate tectonics are due to blobs left behind by cosmic collision

Every drop counts: New algorithm tracks Texas's daily reservoir evaporation rates

Study suggests heavy snowfall and rain may contribute to some earthquakes

Study reveals new mechanism to explain how continents stabilized
Let us know if there is a problem with our content.
Use this form if you have come across a typo, inaccuracy or would like to send an edit request for the content on this page. For general inquiries, please use our contact form . For general feedback, use the public comments section below (please adhere to guidelines ).
Please select the most appropriate category to facilitate processing of your request
Thank you for taking time to provide your feedback to the editors.
Your feedback is important to us. However, we do not guarantee individual replies due to the high volume of messages.
E-mail the story
Your email address is used only to let the recipient know who sent the email. Neither your address nor the recipient's address will be used for any other purpose. The information you enter will appear in your e-mail message and is not retained by Phys.org in any form.
Newsletter sign up
Get weekly and/or daily updates delivered to your inbox. You can unsubscribe at any time and we'll never share your details to third parties.
More information Privacy policy
Donate and enjoy an ad-free experience
We keep our content available to everyone. Consider supporting Science X's mission by getting a premium account.
E-mail newsletter
Laser powder bed fusion of NdFeB and influence of powder bed heating on density and magnetic properties
- ORIGINAL ARTICLE
- Open access
- Published: 29 April 2024
Cite this article
You have full access to this open access article
- Kübra Genç ORCID: orcid.org/0009-0008-7977-4469 1 ,
- Sirapob Toyting 3 ,
- Enrique Galindo-Nava 4 ,
- Iain Todd 2 &
- Kamran Mumtaz 1
197 Accesses
Explore all metrics
Laser powder bed fusion (L-PBF) is an additive manufacturing technique that provides an opportunity to create complex NdFeB magnets, potentially enhancing their performance. L-PBF possesses its own processing challenges, such as porosity/cracks and thermal stresses due to rapid cooling. This study focused on optimizing the parameters and the use of elevated temperature (300–550 °C) powder bed heating to reduce defect generation. This paper includes a detailed process parameter investigation, which revealed samples with a maximum energy product, (BH) max , of 81 kJ/m 3 (remanence, B r 0.72 T; coercivity, H ci 891 kA/m) without post/pretreatment, which are the highest (BH) max and B r for L-PBF-processed NdFeB commercial powder. It was observed that all the high-magnetism samples possessed high density, but not all the high-density samples possessed high magnetism. The SEM images and discussions are academically valuable since they clearly illustrate grain formation and morphology in the melt pool, areas where the literature provides limited discussion. Furthermore, this paper incorporates quantitative phase analyses, revealing that the magnetic properties increase with increasing volume fraction of the strong magnetic phase Nd 2 Fe 14 B. Another significant contribution of this paper is that it is the first study to investigate the effect of heated bed on L-PBF-NdFeB alloys. The density of the samples and B r can be improved with the use of elevated powder bed heating, while the H c decreases. The (BH) max can also be improved from 55 to 84 kJ/m 3 through elevated powder bed heating. The maximum magnetic properties obtained with the heated bed (400 °C) were as follows: B r , 0.76 T; H ci , 750 kA/m; and ( BH) max , 84 kJ/m 3 .
Similar content being viewed by others
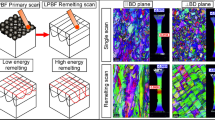
Tailoring Microstructure and Mechanical Properties of Additively Manufactured Inconel 625 by Remelting Strategy in Laser Powder Bed Fusion
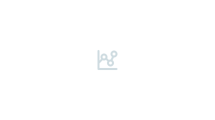
Multi-material additive manufacturing-functionally graded materials by means of laser remelting during laser powder bed fusion
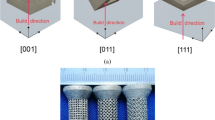
Electron beam powder bed fusion additive manufacturing of Ti6Al4V alloy lattice structures: orientation-dependent compressive strength and fracture behavior
Avoid common mistakes on your manuscript.
1 Introduction
NdFeB magnets are among the most commonly used permanent magnets and are commonly found within electric motors. Due to their high coercivity H c , high remanence B r , and high energy product ( BH) max , they are therefore highly desirable for use within electric motor applications [ 1 ] and can retain their magnetism for long periods of time. However, eddy currents occur in the magnetic core during the operation of magnetic devices, which can cause a temperature increase within the magnet, subsequently reducing its magnetic performance [ 2 , 3 ]. Due to the low Curie temperature of NdFeB magnets, they are sensitive to eddy currents; isolated-segmented permanent magnets are designed to avoid/reduce eddy current losses by decreasing the eddy current path with isolation layers [ 4 ]. With the use of more complex shaped magnets, efficient cooling channels can be integrated to reduce temperature build-up and prevent unnecessary eddy current losses within the magnet [ 5 ]. In addition to cooling channels, the enhancement of magnet efficiency is achievable through shape design, wherein the geometric configuration significantly impacts magnetic properties. Specialized designs have been developed to optimize key features such as magnetic field strength and distribution. The overall shape and dimensions of a magnet play a crucial role in influencing its magnetic strength. For instance, manipulating the length of a magnet while maintaining a constant cross-sectional area can result in an improved magnetic field. In pursuit of intricate magnet designs , research has been conducted analyzing four distinct shapes: fan-shaped, arc-shaped, ring-shaped, and a self-proposed electromagnet. The findings indicated that arc-shaped magnets exhibited the highest field intensity, measuring 0.6 Tesla [ 6 ]. Similarly, other research revealed that triangular magnets outperform trapezoidal or skewed-shaped counterparts in terms of the induced electromotive force and output power in axial flux permanent magnet synchronous generators [ 7 ]. Additionally, investigations have demonstrated a 50% increase in motor output power with V-shaped magnets, with the potential for further enhancements using curved-shaped magnets [ 8 ].
There are a number of production methods capable of manufacturing NdFeB magnets, and the most common are sintering, hot deformation, and injection molding [ 9 ]. However, with these conventional manufacturing techniques, it can be challenging to produce complex/intricate-shaped NdFeB magnets. The most common production method for NdFeB magnets is sintering. It is a powder metallurgy-based process involving powder blending, compaction, subsequent sintering in a furnace, machining, and surface treatment and magnetization [ 10 ]. In contrast, additive manufacturing (AM) is a layer-by-layer additive process in which a magnet is built from powder or wire feedstock. With its slower cooling rates, sintering leads to larger grain sizes in the final magnet, while AM, with higher cooling rates, produces finer grain sizes [ 8 ] that may impact magnetic properties[ 11 ]. In terms of complexity, sintering is limited due to traditional molding and pressing methods, whereas AM allows for intricate and complex geometries. Sintering generates material waste through machining processes for final shapes, while AM generally produces less waste as the material is selectively deposited. Additionally, in sintering, magnetization typically occurs after the process, exposing the magnet to a strong external magnetic field [ 10 , 12 , 13 ]. In AM, magnetization can be integrated into the process or performed afterward. On the other hand, injection molding (IM) is the most widespread method for producing polymer-bonded magnets. Although the injection molding (IM) technique enables the production of complex designs, polymers are mixed within these injection-molded NdFeB magnets, subsequently reducing their magnetic performance [ 14 , 15 ]. Machining can also be used as an option for integrating complexity within a component’s geometry; however, this approach is not viable due to the brittle structure of NdFeB.
Additive manufacturing (AM) presents an opportunity to create complex geometries from various feedstocks. Fused deposition modeling (FDM) [ 16 ], big area additive manufacturing (BAAM) [ 17 ], binder jetting [ 18 ], and powder bed fusion [ 19 , 20 , 21 , 22 ] are AM techniques that have thus far been used for the processing of NdFeB. With polymer-based AM techniques (e.g., binder jetting) for the indirect production of NdFeB components, low magnetic properties are generated due to the inclusion of polymers within the feedstock, reducing the volume fraction of the magnetic structure and creating porosity within the component after the curing of the polymer binder. Previous results have shown that the density of binder jetted magnets is nearly half that of sintered magnets, 3.54 g/cm 3 [ 18 ] and 7.5 g/cm 3 (Arnold Magnetic Tech), respectively. Additionally, when the binder jetted magnet is compared with conventional polymer-based magnets, compression-bonded magnets, and IM magnets, the remanence of the binder jetted magnets is lower than that of both the IM and compression-bonded magnets (binder jet magnet, 0.3 T; IM magnet, 0.5 T; compression-bonded magnets, 0.65 T) [ 14 ]. FDM produces parts with a lower density than IM and sintered magnets; the density percentage of the FDM sample is 3.57 g/cm 3 while that of the IM sample is 4.35 g/cm 3 [ 16 ] and that of the sintered magnet is 7.5 g/cm 3 . The remanence of the FDM-printed sample is 0.31 T [ 16 ], that of the IM sample is 0.38 T [ 16 ], and that of the sintered magnet is 1.2 T (by Arnold Magnetic Tech). The magnetic properties of the parts produced using BAAM are greater than those of the parts produced using the IM magnet by a narrow margin but still less than those of the sintered magnets (BAAM magnet B r = 0.51 T, IM magnet B r = 0.48 T [ 17 ], sintered magnet B r = 1.2 T by Arnold Magnetic Tech).
Direct processing of NdFeB without the need for a binder can be achieved using powder bed AM techniques that employ a high-energy scanning source to selectively melt the powder. This scanning source can either be a laser or an electron beam; however, there is a risk of smoking using an electron, meaning that electron beams charge the powder and displace it from the powder bed. This may not only affect the melting quality but also elevate the powder within the build chamber, which can damage the electron melting system [ 23 ]. Many powders, including Ti–Al [ 24 ], Inconel alloys [ 25 ], and the rare earth permanent magnet Mn-Al [ 26 ], are at risk of smoking. Hence, the powder needs to be preheated and sintered before fully melting with an electron beam, inhibiting powder jumping [ 24 , 25 , 26 , 27 ].
Alternatively, lasers can be used to process the material within the powder bed using L-PBF. The laser powder bed fusion processing parameters for NdFeB powder were investigated, and it was found that high-density parts (with a relative density of up to 86%) can be obtained by the energy line (laser power W/laser speed mm/s) between 0.032 and 0.048 W/mm [ 28 ]. Various L-PBF scan parameters, such as the laser power (LP), laser focus ( LF ), point distance ( PD ), exposure time ( ET ), and hatch distance ( HD ), and their effects on the properties of processed NdFeB parts were studied by [ 29 ]. During processing, the samples were built on a steel substrate plate that was connected to a larger copper substrate. The copper substrate acted as a heat sink during laser-generated heat buildup within the powder bed and built platform. The results show that the grain sizes within the samples were reduced to 1 µm, which is smaller than those produced using commercial sintered magnets. It was found that high cooling rates are essential for obtaining a Nd 2 Fe 14 B phase and fine grains that also increase coercivity. The maximum properties achieved were H ci , 695 kA m −1 ; B r , 0.59 T; and (BH) max , 45 kJm −3 . The density of the produced magnet was 7 g/cm 3 , which is 92% of the theoretical density [ 29 ]. Moreover, it was suggested that the processing window for NdFeB is narrow and that a small change in laser power can cause destabilization of the melt track. In addition, the powder layer thickness must be adequate to achieve a high relative density of the samples [ 21 ]. Bittner et al. had been able to generate some of the highest magnetic properties using L-PBF with a coercivity of 886 kA/m, a remanence of 0.63 T, and a density of up to 90%. Stable processing was achieved with an energy density between 0.6 and 2.3 J/mm 2 [ 19 ].
Moreover, magnetic properties can be enhanced by element intergranular addition, which is one of the methods used to improve the coercivity of sintered magnets [ 30 , 31 , 32 , 33 , 34 ]. An intergranular addition of a low melting point eutectic alloy (Pr 0.5 Nd 0.5 ) 3 (Cu 0.25 Co 0.75 ) was used for SLM-produced NdFeB. The results show that the coercivity of the samples improves with the presence of a low-melting paramagnetic alloy between the Nd 2 Fe 14 B phase grains [ 35 ]. On the other hand, heat treatment is a post-processing method known to enhance the coercivity of sintered magnets [ 36 , 37 ], and it has been reported that it increases the magnetic properties of SLM-NdFeB-printed magnets as well [ 38 ] (where the samples were printed with “sintered magnet alloy” and “near-stoichiometric alloy” powders, which were specifically prepared for this study). Furthermore, the use of a copper-rich NdFeB alloy has been found to improve the coercivity of SLM-ed magnets [ 39 ]. The highest coercivity of SLM NdFeB magnets was reported in this study, with the help of heat treatment. The double scanning technique is another method for improving density and magnetic properties by reducing voids and cracks. The results indicate that the second scan with half laser power after a full power scan does not cause undesirable phase formation [ 40 ] On one side, laser powder bed fusion (LPBF) systems equipped with single and three-beam lasers were employed to produce NdFeB components. This study investigated various process parameters, including the type of laser (pulsed or continuous wave), and their impact on magnetic properties. Utilizing the synchronized three-beam method with a two-zone scanning strategy resulted in an increase in the maximum magnetic energy product of 33.42 kJ/m. 3 . Furthermore, the processing time substantially decreased by 38% compared to that of the single-beam method [ 41 ].
On the one hand, a specific real-life application of laser powder bed fused (L-BPF) NdFeB magnets has not yet been extensively documented in the literature. However, the adoption of L-PBF for NdFeB magnets is an area of ongoing research and development. It is known that permanent magnets, such as NdFeB, can be incorporated into the rotor to create a magnetic field [ 1 ] Hence, the potential applications of L-PBF-produced NdFeB magnets include electric motors. The use of NdFeB magnets with cooling channels [ 8 ] could be one of the potential uses of L-PBF-produced NdFeB in electric motor applications. AM has the potential for the integration of magnets into rotor structures with precise alignment, contributing to improved magnetic flux and motor efficiency. It is well known that customized designs and the ability to optimize the distribution of magnetic materials by AM enhance the performance of motors [ 42 ].
Additionally , it has been reported that AM methods are more cost-effective than traditional methods, particularly for small-batch production. The production volume is a significant independent factor, while customization and complexity are considered interchangeable in terms of their impact [ 43 ]. More specific studies indicate that magnets produced using Big Area Additive Manufacturing (BAAM) exhibit superior cost-effectiveness compared to injection-molded magnets, with comparable or even enhanced performance [ 44 ]. However, there is a lack of specific cost-effectiveness studies on laser powder bed–fused NdFeB magnets. Nevertheless, considering the known advantages of AM technology, such as design complexity without additional tool or machining costs and minimal material waste, it is anticipated that L-PBF holds potential as a cost-effective method for producing NdFeB magnets.
Table 1 compares the magnetic properties of AM and the sintered magnets. L-PBF-processed NdFeB is superior to polymer-based AM techniques in terms of density and magnetic properties, while it is inferior to sintered magnets. Increasing the volume fraction of magnetic powder (f) may improve the magnetic properties since the energy product, ( BH) max , is proportional to f 2 [ 18 ].
The origin of the high remanence of the NdFeB magnet is the highly anisotropic 2:14:1 tetragonal phase (in this case, Nd 2 Fe 14 B), called the hard magnetic phase and represented by θ . This phase has a high saturation magnetization and anisotropy constant, which results in high magnetization. To obtain the optimum magnetic performance, fine and homogenously distributed Nd 2 Fe 14 B phases surrounded by an amorphous phase, which isolates adjacent grains and ensures high coercivity, are needed [ 45 ]. Therefore, it is important to develop optimum AM processing conditions to attain this desired microstructure. In this study, L-PBF was used to process NdFeB powder, and the main objective is to improve the density of L-PBF-printed magnets, reduce defects, and understand the effect of density and pre-heating on magnetic properties.
Preheating and rescanning reportedly reduce residual stresses in L-PBF processing of standard alloys (e.g., 316 L stainless steel [ 50 ] and Ti6Al4V [ 51 ]); thus, the use of pre-heating is examined in this paper. The expectation is that a reduction in cracks and porosity within NdFeB samples can be achieved by reducing thermal stresses, with the potential to alter and improve magnetic properties.
2 Materials and methods
This study used a commercial NdFeB powder with a spherical morphology sourced from Magnequench (MQP-S-11–9-20,001). Powders were L-PBF processed using an Aconity Mini (for preliminary experimentation) and an Aconity Lab (for elevated temperature powder bed heating). Both systems use a 200-W continuous-mode laser with a 1070 nm wavelength and 50 µm and 80 µm laser spot sizes. Cubic samples (10 × 10 × 10 mm) were processed under a purged argon atmosphere (maintaining oxygen levels below 200 ppm). For the powder bed heat experiments, the samples were processed using the Aconity Lab L-PBF system. Powder bed temperatures were set between 300 and 550 °C in 50 °C increments with oxygen levels below 50 ppm. The temperatures of the powder bed were checked using a gun pyrometer. The densities of the samples were analyzed using the Archimedes density test in water. The morphology of the grains was analyzed using a Nikon optical microscope after etching using nital to reveal the grain boundaries. Phase analysis was performed using an XRD system, PANalytical X’Pert 3 (reflection). The magnetic properties were tested using a Helmholtz coil-fluxmeter and a permeameter by Arnold Magnetic Technologies. The internal porosity analysis was performed using a Micro CT (Nikon Metrology XTH 225/320 LC). SEM analysis was performed using Inspect F and Inspect F50.
2.1 Powder characterization
Commercial magnetic NdFeB powder (MQP-S-11–9-20,001) possessed particle sizes of d 10 = 20.1 µm, d 50 = 41.1 µm, and d 90 = 70.3 µm (measured using a Mastersizer 3000 laser diffraction analyzer). Figure 1 shows the powder morphology, and Table 2 shows the composition of the powder.
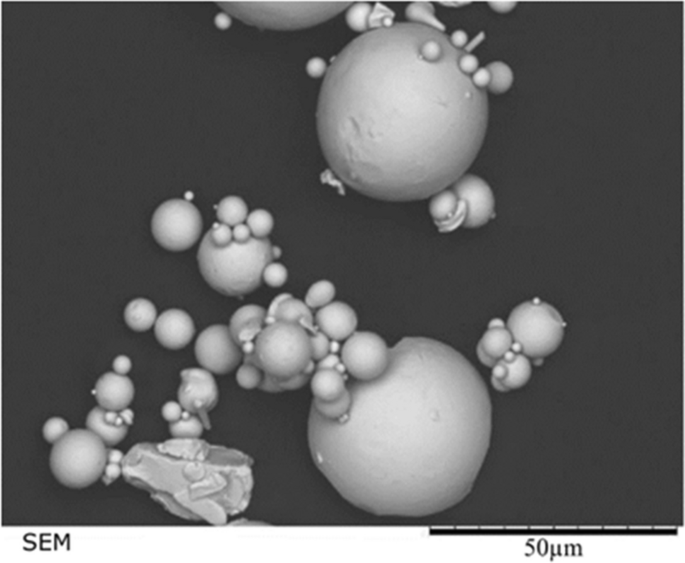
SEM image of the NdFeB powder MQP-S-11–9-20,001
2.2 Experimental parameters
In this study, the L-PBF parameters laser power ( LP ), laser speed (LS), hatch distance ( HD ), layer thickness (LT) , and energy density (ED = LP/(LS*HD*LT)) were studied and optimized to attain high-density defect-free components. The experimental setup comprises two stages. The primary objective of the first stage is to identify relatively safe combinations of laser power and laser speed and, subsequently, safe EL while keeping the LT and HD fixed. This approach is taken before delving into more detailed process parameters. The intention is to establish a foundational understanding in stage 1 before progressing to more comprehensive analyses in stage 2.
In the first stage, the processing parameters were set based on published papers by Urban et al. [ 46 ] and Kolb et al. [ 28 ]. The former paper identified that samples with laser power ( LP ) ranging from 40 to 55 W and laser speed ( LS ) from 1200 to 2000 mm/s exhibited higher magnetic polarization, with a LT and HD of 20 µm. Furthermore, their density results indicated that a laser power within the range of 40–55 W and a laser speed between 1000 and 3000 mm/s resulted in relatively high densities. Higher laser powers up to 90 W were also investigated. Consequently, for the initial stage of the experiment, it was decided to set the laser speed between 1000 and 3000 mm/s and the laser power from 40 to 100 W, with a fixed LT of 20 µm, as per the published paper. The HD was fixed at 30 µm due to early experimental failures observed with the combination of 20 µm LT and 20 µm HD . All combinations of first-stage process parameters set with LP between 40 and 100 W; LS between 1000 and 3000 mm/s; LT , 20 µm; and HD , 30 µm are detailed in the Appendix. On the other hand, the latter paper indicated that an energy density ( EL ), calculated as LP/LS , between 0.03 and 0.05 Ws/mm [ 28 ] yielded the highest relative density. Therefore, only process parameters within this EL range were utilized in the first stage, as shown in the Appendix.
In the second stage, the parameter design was based on the outcomes of the first stage. In this stage, experiments were broadened to investigate the effect of HD , in addition to investigating the effect of higher laser powers up to 200 W and wider laser speed range from 1500 to 5500 mm/s, in addition to a wider energy density range of 20–130 J/mm 3 .
A summary of the printing parameters is shown in Table 3 .
3 Results and discussion
3.1 preliminary experiment, first stage.
There was no successful printing below a laser power of 60 W, as shown in Fig. 2 a (samples 1 to 5). It is assumed that the low laser power (below 60 W) was insufficient to melt the NdFeB powder. Interestingly, the failed samples from 1 to 5 ( LP , 40–50 W) in Fig. 2 a have the same ED as the successfully printed samples near them. Despite all the EDs being between 50 and 83 J/mm 3 , the only successful printing was obtained with an LP of 60 W and above. Additionally, the damage of the samples (samples 6 to 9) is due to the failure of nearby samples. This is because the broken pieces and layers from the failed samples (samples 1 to 5) are carried onto these samples by a wiper during powder layer deposition. Sample 6 and sample 8 were repositioned and reprinted successfully, as shown in Fig. 2 b.
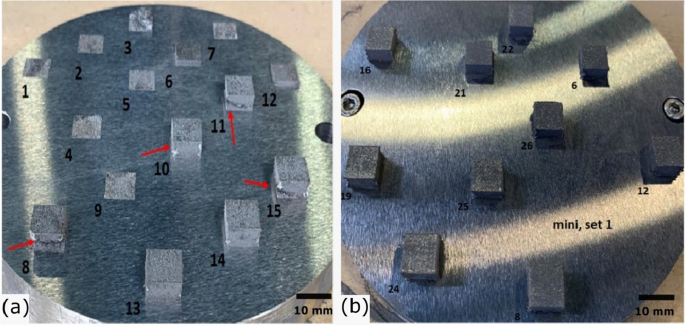
Processed samples using Aconity mini, LP a samples 1–2; 40 W; samples 3–5, 50 W; samples 6–9, 60 W; samples 10–13, 70 W; samples 14–15, 80 W; b sample 16, 80 W; samples 19–22, 90 W; samples 24–26, 100 W. ED 50–83 J/mm. 3 , scan parameters can be seen in Table 4 (red arrows indicate defects) (samples 17–18–20 and sample 23 were not printed due to software errors)
Some defects are observed on the corners and the bottom of the samples (shown by the red arrows in Fig. 2 a). It is possible that the temperature differences between the cold building platform and the hot melted powder cause thermal stresses and subsequently cause these defects and cracks on the bottom of the samples. As the printing progresses, the new layers are melted on the previously melted hot layer. The temperature differences between the layers decrease as the building progresses, reducing the thermal stresses on the top part of the samples. Table 4 shows the detailed process parameters for the samples produced in Fig. 2 . The process window is LP from 40 to 100 W in 10-W increments, HD 30 µm and LT 20 µm.
Figure 3 a shows that the density of the samples increases with increasing laser energy density. The highest density was achieved using 100 W, 95.72%, sample 24, while sample 6 had approximately the same energy density as sample 24 but used 60 W LP , generating a 91.52% sample density. Both of these samples are indicated with red circles in Fig. 3 a, and their micro CT scan is shown in Fig. 4 . It can be seen that the higher LP samples have higher densities than the lower LP samples in the same energy density range. Another important aspect worth noting is the pronounced effect of laser power on the part density. Varying the laser power affects the minimum energy density required for achieving 91% dense NdFeB L-PBF parts. Figure 3 a illustrates an inverse relationship between LP and the minimum ED needed for the effective melting of NdFeB powder in the L-PBF process. At 60 W LP , a minimum ED of 80 J/mm 3 is needed, whereas at 80 W LP , the minimum ED required to achieve 91% dense parts decreases to 59 J/mm 3 . This suggests that the laser power has a more significant impact on NdFeB melting in the laser powder bed fusion process. The processing quality is more likely dependent on the laser power; however, increasing the ED also increases the density of the samples, as shown in Fig. 3 a. Figure 3 b shows a similar trend for the magnetic remanence values. Higher remanence values were achieved using higher laser powers. The maximum B r , 0.658 T, was obtained using 100 W (sample 24, red circle in Fig. 3 b), while the B r value of sample 6 is 0.591 T, red circle in Fig. 3 b. Sample 6 was processed using 60 W of LP and contains more lack of fusion (LoF) porosities than sample 24 (as shown in Fig. 4 a and b), while their HD and LT remain the same (the energy densities are nearly the same, 74 J/mm 3 and 80 J/mm 3 ). These LoF porosities reduce the percentage of magnetic structure in the components and hence reduce the B r . Additionally, two main phases were detected in sample 6 and sample 24, namely soft magnetic α-Fe and hard magnetic Nd 2 Fe 14 B, as shown in Fig. 4 c. It is assumed that the greater amount of the a -Fe phase in sample 6 reduces the B r content by reducing the volume percentage of the hard magnetic phase and because of the soft magnetic structure of α-Fe [ 29 ]. The greater amount of the a -Fe phase and the lower amount of the Nd 2 Fe 14 B phase in sample 6 are likely due to the low LP / LS ratio, which causes lower cooling rates.
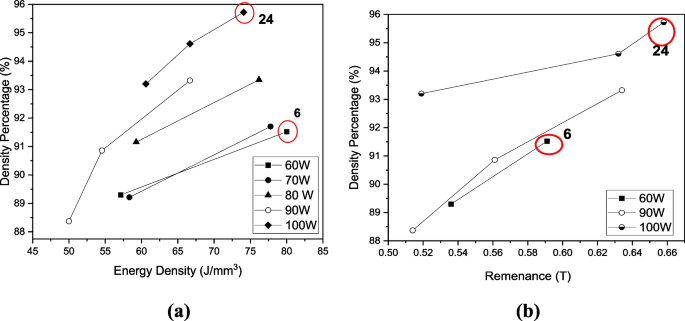
a Relationship between the energy density, sample density, and laser power; b relationship between sample density, remanence, and laser power
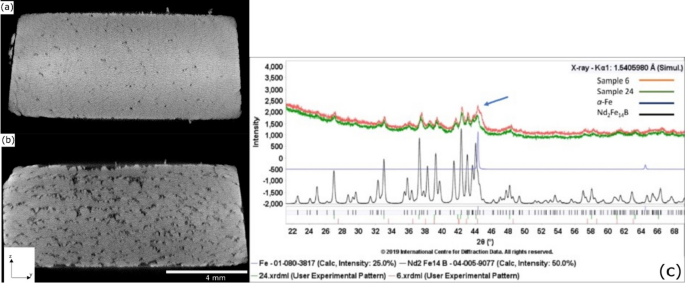
Micro-CT images; a sample 24, LP 100 W– ED 74 J/mm 3 and b sample 6, LP 60 W– ED 80 J/mm 3 , side view of the samples; c XRD patterns of printed samples 6 and 24, where the blue arrow points to the α-Fe phase
The first stage of the experiments was used to narrow the process parameter window to reduce the risk of failure and to control the effect of the laser power and laser speed on both the density and magnetic properties. In the second stage, the process parameter window was widened based on the preliminary results.
3.2 Effect of laser power and energy density on sample density
As the laser power increases to 100 W, the density increases, as identified within the preliminary experiments. However, further investigations on the laser power show that the density of the samples starts to decrease after 120–130 W despite the energy densities being within the same range as those of the lower laser power samples, as shown in Fig. 5 . The reason for this reduction in sample density may be the result of the higher scan speeds. The combination of high laser speed and high laser power generates unstable melt pools that result in low-density samples. It is also worth noting that increasing the laser power above 130 W may cause excessive laser energy exposure, causing overheating of the powder and the creation of keyholes within the melt pool; hence, the sample density decreases.
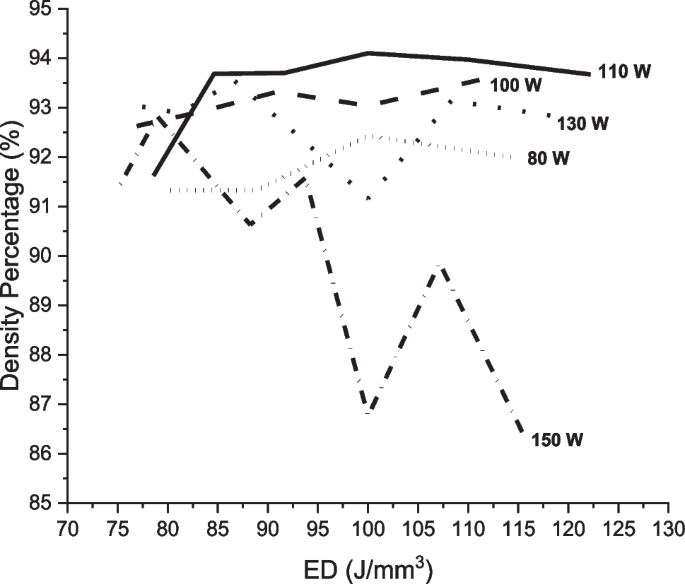
Relationships between the ED and density of the samples with various laser powers (80–150 W, HD 20 µm– LT 20 µm)
The laser speed should be low enough to allow sufficient melting and powder consolidation, and the laser power should be high enough to melt the powder but needs to be low enough to avoid overheating the powder. In this study, the successful laser power range is defined between 60 and 130 W, based on the results in Figs. 2 and 5 .
Moreover, no sample could be produced above 125 J/mm 3 due to the excessive energy density causing material evaporation. High energy densities created keyholes within the melt pool, creating extremely brittle samples. It is essential to maintain the energy density below the maximum critical ED value, 125 J/mm 3 , to avoid keyhole pores. In addition, high EDs cause higher residual stresses within the sample [ 30 , 31 , 52 , 53 ] The combination of tensile stresses occurs on top of the samples due to the material shrinkage caused by rapid cooling, and the compressive stresses on the bottom of the sample [ 34 , 54 ] cause cracks [ 47 ] and consequently material delamination. It is essential to maintain the energy density below the critical ED value (in this study, 125 J/mm 3 ) to reduce cracks, delamination, partial distortion [ 47 ], and keyhole pores. However, it is also necessary to generate sufficient energy density to prevent a lack of fusion porosity.
Figure 6 a shows that the high-density sample (95.72%) uses an ED 74 J/mm 3 while Fig. 6 b shows the lack of fusion pores in the low ED sample ( ED 50 J/mm 3 , sample density percentage 88.37%). Additionally, the very high LP /fast LS combination (200 W/5500 mm/s) negatively impacts the geometric integrity of the sample, as shown in Fig. 7 a and b.
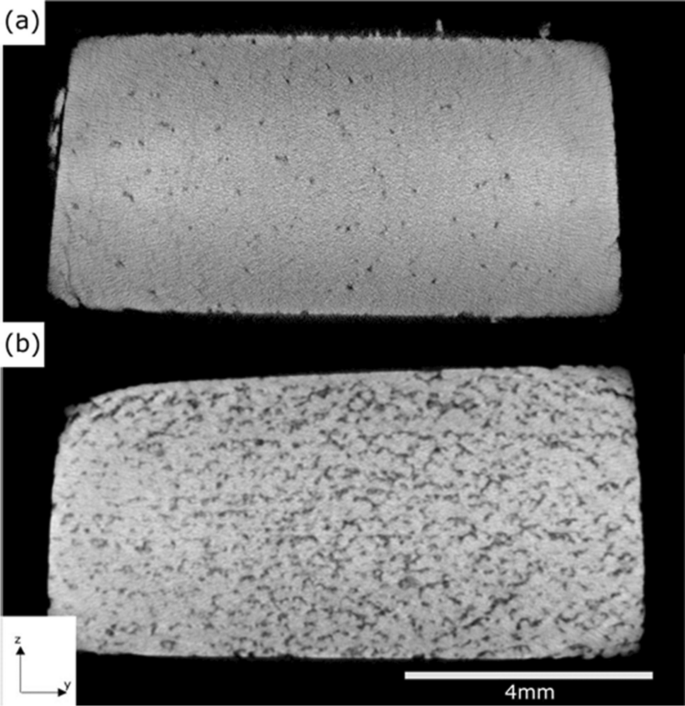
Micro-CT scans of a sample 74 J/mm 3 (100 W, 2250 mm/s, 95.72%) and b sample 50 J/mm. 3 (90 W, 3000 mm/s, 88.37%)
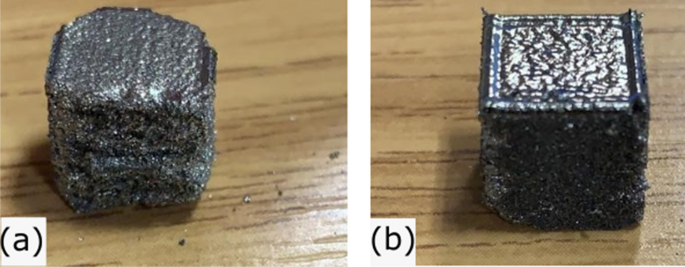
a 90.9 J/mm 3 (200 W, 5500 mm/s, 20 µm HD 20 µm LT ) and b 92.85 J/mm 3 (140 W, 3500 mm/s, 20 µm HD , 20 µm LT ), sample size 10 mm × 10 mm × 10 mm
3.3 Magnetic test results/relationship between density and remanence
The densities of the 78 samples plotted with respect to LP from 70 to 200 W and LS from 1500 to 5000 mm/s are shown in Fig. 8 a. Since the energy density takes into account four independent process parameters \((ED=LP/(LS*HD*LT),\) the HD and LT were kept constant. The red areas represent regions of high density within the samples. The results show that the maximum obtained density was 7.066 g/cm 3 , which is 94%, based on the theoretical density of NdFeB magnets, which is 7.5 g/cm 3 . Furthermore, the remanence values of 57 of the 78 samples were tested by a Helmholtz coil and fluxmeter, and the results are plotted in Fig. 8 b. The arrows in Fig. 8 b show the direction of increasing scan speed; consequently, the solidification process is faster, resulting in higher remanences. The results correspond to published results by J. Jaćimović et al. [ 29 ]. Generally, the remanence increases as the sample density increases, as shown in Fig. 8 c. All the high magnetic samples have a high density; however, not all the high density samples are highly magnetic. Figure 8 d shows that higher B r values are obtained for ELs between 0.03 and 0.04 W/mm with EDs between 75 and 100 J/mm 3 . The B r value decreases to 0.03 T by increasing EL to 0.05 Ws/mm and ED to 125 J/mm 3 . It is concluded that the magnetic properties are related not only to the density of the samples but also to the cooling rate. The following section will discuss in more detail the analysis of the specific parameter contributions to the phases and magnetic properties.
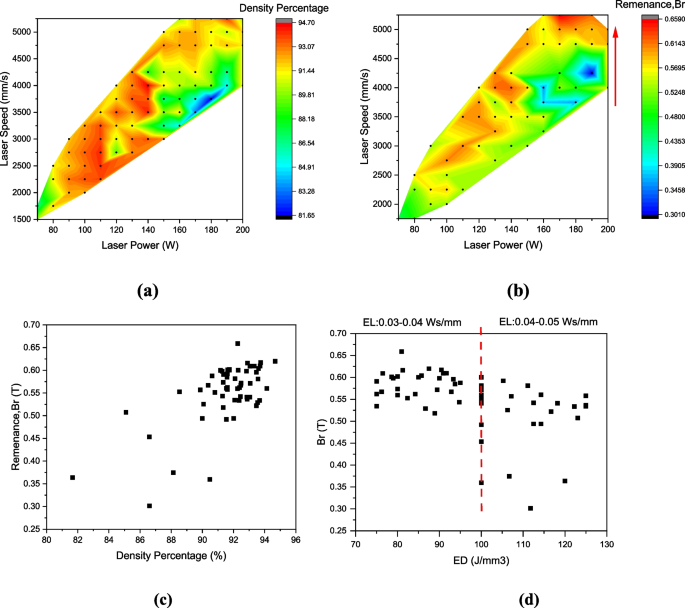
a The Archimedes density results of the samples as a function of the laser speed and laser power; b the remanence of the samples, tested by a Helmholtz coil and fluxmeter, as a function of the laser speed and laser; c the dependence of the remanence on the density percentage of the samples; d the dependence of the remanence on the energy density, where the red line is the 0.04 Ws/mm EL , ( HD , 20 µm and LT , 20 µm)
3.4 Relationship between the phase and magnetic properties
The maximum energy product, (BH) max , is one of the essential magnetic properties used to evaluate the performance of permanent magnetic materials. It is the maximum BH value in the second quadrant of the hysteresis loop [ 35 ]. Thus, the remanence, B r , needs to be considered to improve the magnetic performance. In our case, the XRD analyses show that the remanence, B r , increases as the volume fraction of the Nd 2 Fe 14 B phase increases, as shown in Fig. 9 a. The reason for this increase is the high saturation magnetization of the Nd 2 Fe 14 B phase, which improves the remanence [ 55 ]. Coercivity is the resistance to demagnetization of the magnet, and thus, it is the other important magnetic property to assign to high-performance magnets [ 56 ]. High magnetocrystalline anisotropy is one of the requirements for high coercivity. Low magnetocrystalline anisotropy is observed in high-symmetry systems, such as Fe, which means that there are many easy axes in the structure, and magnetic domains are easily aligned in many different directions by an external field. However, in less symmetrical systems with high magnetocrystalline anisotropy, such as the tetragonal Nd 2 Fe 14 B phase, there are only two axes whose domains can easily align. In this phase, more energy is needed to change the domain structure. Therefore, magnetic domain motion is restricted, preventing decoupling between domains, which improves coercivity [ 55 ]. Graph b in Fig. 9 shows the increase in the intrinsic coercivity, H ci , value with increasing volume fraction of the Nd 2 Fe 14 B phase, possibly resulting from the high magnetocrystalline anisotropy of the Nd 2 Fe 14 B phase. There is a clear increasing trend in the B r value with increasing volume fraction of the Nd 2 Fe 14 B phase, as shown in Fig. 9 a, while there is a decreasing trend in the B r and H ci values with increasing volume fraction of the α ‒Fe phase, as shown in Fig. 9 c and d. This decrease is because the magnetically soft α -Fe phase reduces the amount of the hard magnetic phase, Nd 2 Fe 14 B, and reduces the remanence. Furthermore, since α -Fe is a soft magnetic phase and easily demagnetized, it acts as the center of reversed magnetic domains, lowering the coercivity [ 29 ].
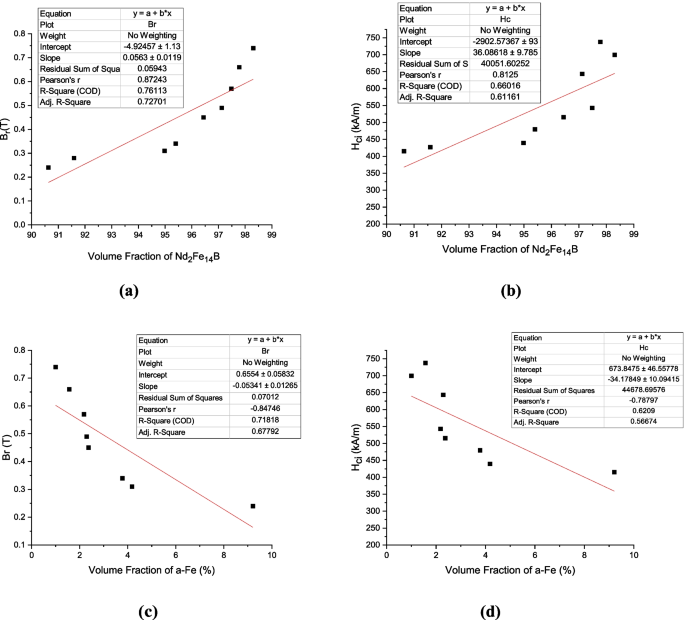
Relationships between a the volume fractions of Nd 2 Fe 14 B and B r ; b the volume fractions of Nd 2 Fe 14 B and H ci ; c the volume fractions of α -Fe and B r ; and d the volume fractions of α-Fe and H c i
The smaller the residual sum of squares is, the better the fit of the data, and the greater the residual sum of squares is, the poorer the fit of the data. The errors of H ci / a -Fe and H ci /Nd 2 Fe 14 B are high. Even for the generally decreasing intrinsic coercivity trend observed with increasing a-Fe phase and decreasing Nd 2 Fe 14 B phase, the relationships between these phases are not linear. Therefore, it is confirmed that H ci is related not only to the phase but also to the microstructure [ 21 ]. On the other hand, even though the fitting is greater in the B r /Nd 2 Fe 14 B and B r / a -Fe graphs, the error values are not 0. This is possibly due to the cracks and porosities within them, which affect the amount of magnetic phase Nd 2 Fe 14 B in the samples.
3.5 Relationship between the phase/laser power and the phase/laser speed
The relationship between the laser power and magnetic properties was investigated for the LP samples from 100 to 180 W with 10-W increments, LS 3500 mm/s, HD 20 µm, LT 20 µm. The results are shown in Fig. 10 . The maximum B r value, 0.74 T, was obtained at LP 130 W, and the highest H c value, 737 kA/m, was obtained at LP 120 W. The decrease in B r and H ci values after 130 W and 120 W, respectively, is similar to that found in the reduction in density of the samples after 120–130 W. The reason behind the decrease in B r and H ci is the decreasing density of the samples due to the high EL , which produces an unstable melt pool in the powder bed. The samples with a 140-W laser power have EL = 0.04 Ws/mm and ED = 100 J/mm 3 , and the samples having higher laser powers than 140 W result in higher EL and ED values, which reduces B r , which supports the results in Fig. 8 d. The trends in the laser power B r and laser power H ci graphs are related to the volume fraction of the Nd 2 Fe 14 B and α -Fe phases. The increase in B r is directly proportional to the increasing volume fraction of the Nd 2 Fe 14 B phase and decreasing α -Fe phase, as shown in Fig. 10 .
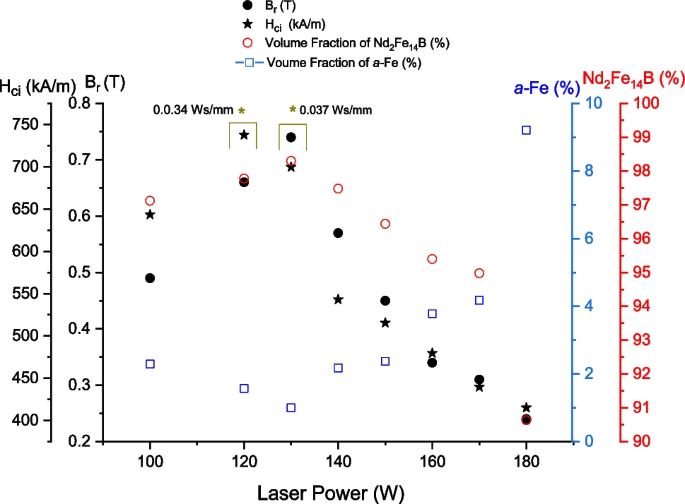
Relationships between the LP , B r , and H ci volume fractions of Nd 2 Fe 14 B and α -Fe, LS 3500 mm/s, LT 20 µm, and HD 20 µm
Figure 11 shows the effect of laser speed on the B r value. B r improves from 0.389 T to 0.622 T with a decrease in LS from 3250 to 2000 mm/s. An increase in LS was expected to improve the B r value since it increases the cooling rate. However, it was noted that the ED is very low and not capable of fully melting the powder . Low EDs cause poor fusion; hence, there is a lack of fusion porosity [ 47 ]. In Fig. 11 , B r improves from 0.389 to 0.622 T by decreasing the laser speed due to the increase in ED from 38 to 62 J/mm 3 . It is also observed that the EL is above 0.04 Ws/mm for the samples with higher B r , 0.52 T, and above. However, they are not negatively affected by the high EL rates, which was expected based on the previously discussed result in Fig. 8 d. This is because the HD of the samples plotted in Fig. 11 is 40 μm, while it is 20 μm for the samples plotted in Fig. 8 d. A high HD causes poor melting characteristics and hence requires a slower scan speed, causing a higher EL to be able to melt the powder.
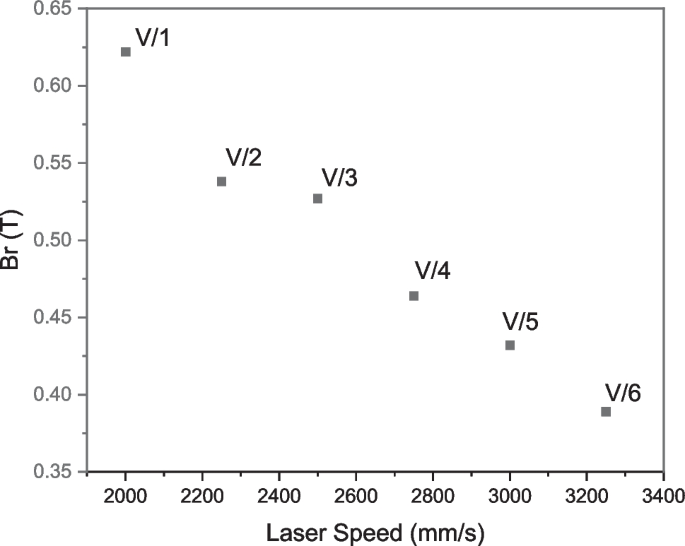
B r and density of the sample with respect to laser speed, HD 40 µm and LT 20 µm, taken by a Helmholtz coil and fluxmeter
It was found that the volume fraction of the Nd 2 Fe 14 B phase is 92% in sample V/3- B r 0.527 T; 90% in sample V/5- B r 0.432 T; and 88.12% in sample V/6- B r 0.389 T, while the volume fractions of a -Fe is 5.28%, 8.08%, and 10.63%, respectively. The greater amount of the Nd 2 Fe 14 B phase in the high- B r samples is shown in the XRD patterns in Fig. 12 . It is known that a high B r content mainly originates from the hard phase [ 21 ]. The density of the samples fluctuates between 90.53 and 93.33%. The maximum B r sample also has the maximum density of 93.33%. The parameters of the analyzed samples are shown in Table 5 . LP was kept constant at 100 W, LS was set from 2000 to 3250 mm/s, HD was 40 µm, and LT was 20 µm.
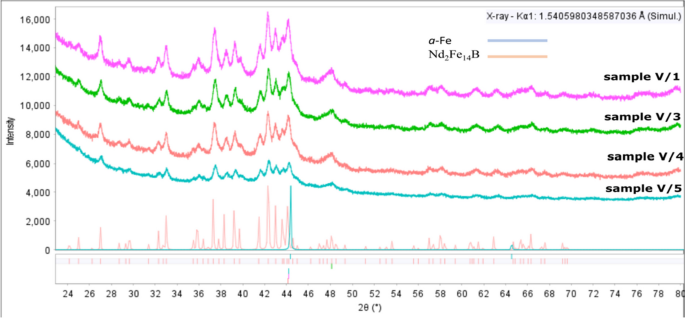
XRD pattern of sample V/1-V/3-V/4-V/5, which is plotted in Fig. 11
3.6 Heated bed
The temperature on the build platform was measured using a thermocouple to ascertain the actual temperature on the build platform before being subjected to the heated bed printing.
The temperature difference between the system (data collected from the thermocouple fitted under the heated bed) and the temperature on the build platform (data collected by the thermocouple connected on the build platform, which is placed on the heated bed) was measured. The thermocouple measurement results are plotted in Fig. 13 a. The difference is small, between 10 and 100 °C, until 380 °C, after which it increases to 160 °C until cooling starts at 75 min.
It was then decided to use a heated bed with low temperatures of 200 °C and 400 °C with the following process parameters: 130 W LP 3500 mm/s, LS 20 µm, LT 30 µm HD to investigate how the system works without compromising the samples at high temperatures. Both temperature trials failed due to the extremely brittle samples being created and due to the incomplete fusion/attachment of the sample to the build platform. The temperatures of the samples were checked using a gun pyrometer. The temperature of the printed sample at 200 °C (thermocouple temperature in the system) was 400 °C (checked by gun pyrometer), and the temperature of the sample processed at 400 °C (thermocouple temperature in the system) was 600 °C (checked by gun pyrometer). Pyrometer measurements were taken from the surface of the samples. Figure 13 b shows the temperature differences between the sample and the system temperature in detail. The temperature difference between the sample and the system was 100 °C at a 380 °C system temperature; then the temperature difference increased to 280 °C by increasing the system temperature to 580 °C. It was concluded that the temperature difference increases with increasing set/system temperature and cannot be fully relied upon.
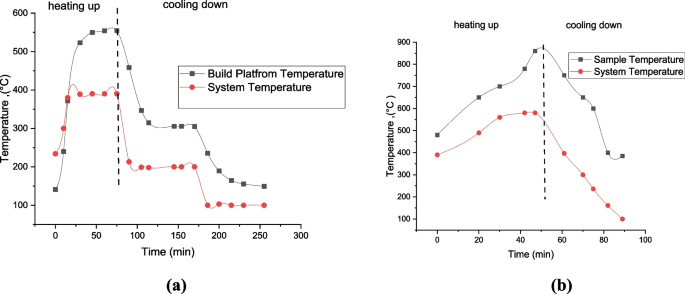
a Build platform temperature vs. time versus software temperature vs. time; b sample temperature vs. time versus software temperature vs. time
The excessive heat within the build platform, generated in combination with the heated bed and laser, made it difficult to produce samples because the first couple of layers did not attach to the build platform. A possible explanation for this may be due to material evaporation caused by excessive heating. The other possibility is that rapid cooling, especially if the bed temperature is too high, can lead to quick and uneven contraction. This can result in stress within the material and affect adhesion to the build platform. It was therefore decided to turn off the heater during the first half of the build to ensure that the samples attached to the platform consistently and did not break due to the powder deposition wiper movement. The heater was then turned on for the second half of the build. The entire building consisted of 335 layers, and the heated bed was turned on at layer 185. A gun pyrometer was used to check the temperature of the samples in the closed chamber after the end of each heated bed trial.
The selected bed temperatures for the subsequent experiments were initially intended to range from 480 to 880 °C, following the heat treatment temperatures for NdFeB magnets in the literature [ 37 ]. However, the temperatures in our study were set between 300 and 550 °C with 50 °C increments since the prior heated bed investigation showed that the sample temperatures were far above the software temperatures: 480 °C for the 380 °C software temperature, 650 °C for the 480 °C software temperature, and 780 °C and 860 °C for the 580 °C software temperature, as presented in Fig. 13 b.
3.7 Effect of the heated bed on the density and magnetic properties of the samples
The heated bed improved the density of the samples from 90 to over 96%. Despite this improvement in density, there is no increasing trend between the rising bed temperature and the sample density. The average density of the samples is 93.93% at 300 °C, 96.66% at 350 °C, 93.80% at 400 °C, 94.60% at 450 °C, 96.4% at 500 °C, and 96.3% at 550 °C (Fig. 14 ). The fluctuations in the densities of the three samples at every bed temperature from 300 to 550 °C are likely related to the instability of the building platform. Figure 15 shows the printed samples at 300 °C and 500 °C. It is deduced that the delamination near the top surfaces and the corners indicated by the red arrows are related to the faster cooling rates on the top of the samples. Printing at higher bed temperatures, such as 550 °C, may introduce greater thermal stress during cooling. The rapid cooling rate or thermal contraction at this temperature could lead to delamination, particularly at the top layers. In contrast, a lower bed temperature, such as 300 °C, may provide a more gradual cooling process, reducing the risk of thermal stress-induced delamination.
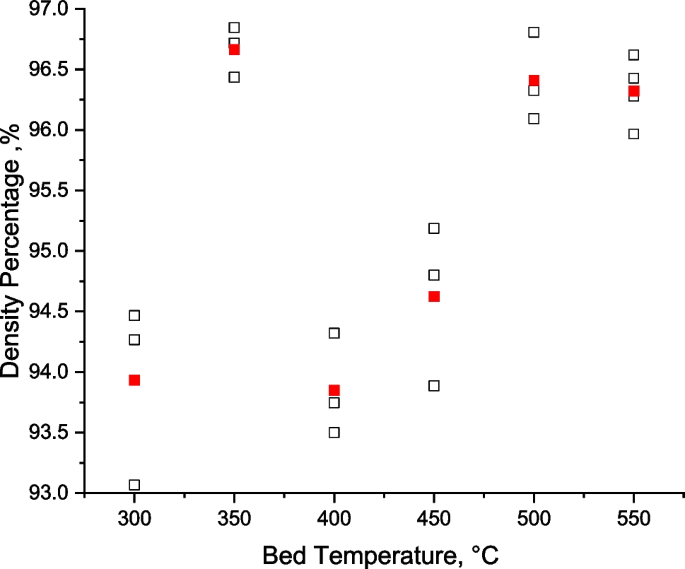
Relationship between the bed temperature and sample density; the red points are the average density of the samples
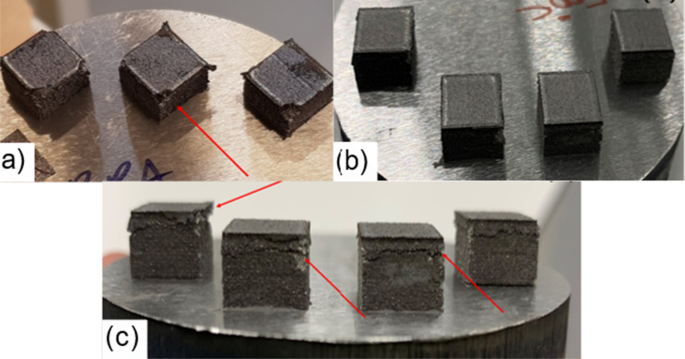
Heated bed-printed samples; bed temperatures at a 300 ℃ (410 ℃); b 500 ℃; and c 500 ℃. The temperatures in the parentheses were checked with a gun pyrometer after the printing finished (sample size: 10 mm × 10 mm × 10 mm); the red arrows point to the cracks and delamination
A clear improvement is observed in the density of the samples produced with the heated bed, as shown in Fig. 16 a. Despite the B r increments not following the density increment trend, the heated bed improves the B r and ( BH) max values, as shown in Fig. 16 a and b. B r increases from 0.61 to 0.76 T, while ( BH) max increases from 55 to 84 kJ/m 3 . Moreover, there is a trend in the ( BH) max . Despite the fluctuations in the B r and H c values, the ( BH) max decreases as the heated bed temperature increases from 400 to 550 °C. Regardless of the decreasing trend in the ( BH) max , it is still superior to that of the room-temperature-printed sample. In contrast, the H c value drops dramatically with the heated bed, from 1000 to below 850 kA/m, as shown in Fig. 16 b. In summary, the increasing heated bed temperature shows no trend in either the B r or H c values, while ( BH) max decreases with increasing heated bed temperature. It is concluded that the heated bed increases the density of the sample, hence increasing the remanence by increasing the percentage of the magnetic content in the entire structure. The heated bed decreases the cooling rate, which is expected to result in a lower percentage of the θ phase in the samples. However, Fig. 16 a shows improvements in the B r values; hence, it is concluded that the effect of density improvement is greater than the effect of lower cooling rates on B r . Even though the heated bed reduces the cooling rates with extra energy input from bed heating, the cooling rates are still much higher than the cooling rate required for the θ phase.
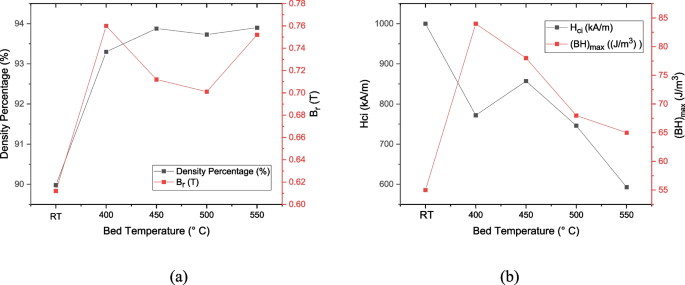
Relationships between a the density percentage of the sample and the heated bed temperature; B r and heated bed temperature; b (BH) max and heated bed temperature; H ci and heated bed temperature. Magnetic tests were performed with a permeameter from Arnold Magnetic Technologies (RT, room temperature proceed sample)
3.8 Microstructural evaluation of heated bed printing
The microstructures of the heated bed samples are different from those of the room-temperature samples. The grain morphology in the room-temperature samples is more globular than that in the heated bed samples, as shown in Fig. 17 . In comparison, the dendritic grains observed in the heated bed samples are mostly oriented in the direction of the building, as shown in Fig. 17 a, b, and c. The decrease in the intrinsic coercivity of the heated bed samples may be related to dendritic grain formation. The lower cooling rates of the heated bed cause in situ heat treatment during and after the build, allowing grains to grow larger, while high cooling rates in the room temperature process result in finer grains. It is known that the smaller grains increase the coercivity [ 14 , 22 ], and it is known from the literature that homogenously distributed grains are needed to improve coercivity [ 57 ]. In addition, irregularly shaped grains and grain boundaries also reduce the coercivity [ 55 ]. Thus, it can be deduced that the dendritic grains and the larger grains in the heated bed samples could be the reason for the decrease in intrinsic coercivity within the samples when using the heated bed. However, B r improved with the use of the heated bed, possibly due to the lower porosity in the heated bed samples.
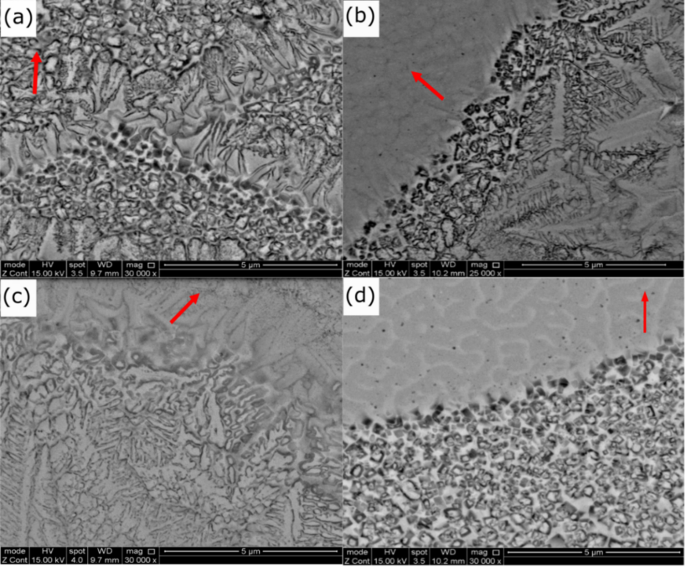
SEM images of the heated bed-printed samples a at 400 °C, b at 450 °C, and c at 500 °C; d the RT-printed sample; the red arrows indicate the z -axis build direction
The microstructural evolution of the NdFeB-heated bed-printed sample is complicated due to the different cooling rates in the melt pool zone (MPZ) and the heat-affected zone (HAZ). Two different grain morphologies were observed in the MPZ and HAZ. Figure 18 shows the top view of the sample at 400 °C. The grains in the MPZ are more spherical and smaller than those in the HAZ. Since the grains in the HAZ were exposed to heat from the previously scanned powder, they had more time to grow. Hence, grain sizes gradually decrease from the bottom of the MPZ to the top of the MPZ, as shown in Fig. 18 d.
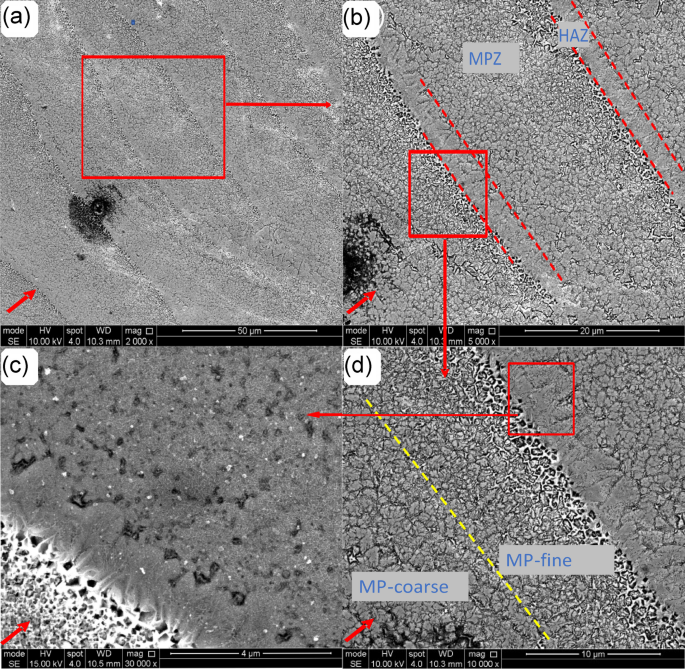
SEM micrographs of the L-PBF - heated bed-printed samples at 400 °C-top view a laser scan tracks of the microstructure; b melt pool zone (MPZ) and heat-affected zone (HAZ); c a closer look at the HAZ; and d coarse and fine grain areas in the MPZ; red arrows indicate the z-axis
Figure 19 shows the cross-section of the 400 ℃ sample. The melt pool lines can be seen in Figs. 19 a and b. A closer look at the HAZ and MPZ shows that equiaxed grains are observed in the MPZ, while columnar grains are observed in the HAZ/melt pool line, as shown in Fig. 19 c and d, respectively.
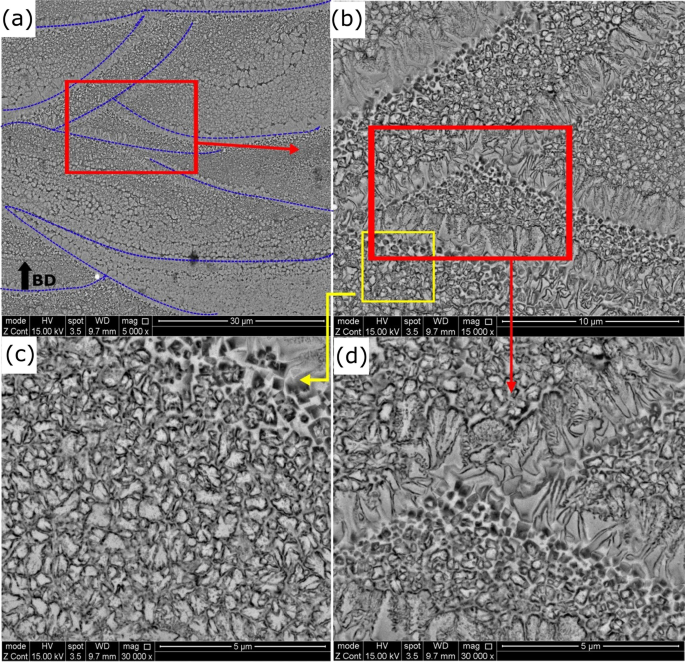
SEM micrographs of L-PBF-heated bed-printed samples at 400 ℃ cross-section: a melt pool lines; b closer evaluation at the melt pool line (HAZ) and melt pool zone (MPZ); c fine grains in the MPZ; and d closer evaluation at the HAZ
In the samples produced at 400 °C, columnar dendritic grains are observed only at the melt pool line (HAZ), and globular and equiaxed grains are observed in the MPZ. A different microstructure is observed in the 500 °C–printed samples. The higher temperatures cause the columnar dendritic grains to be not only in the melt pool line but also in the melt pool zones since the heat-affected area expands into the melt pool zone, as shown in Fig. 20 a and b. The grains in the MPZs are mostly columnar dendrites, where they narrow and cross each other and cause slower cooling rates, which allows for dendritic growth. However, there are still globular/equiaxed grains in the middle of the larger MPZ where the cooling rates are faster, as shown in Fig. 20 c and d.
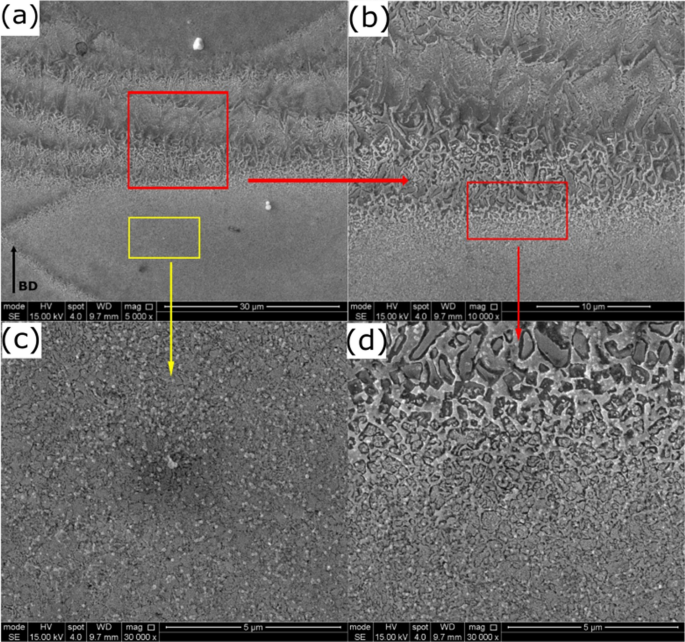
SEM micrographs of L-PBF heated bed samples at 500 ℃ cross-section: a laser scan tracks/melt pool lines on the sample; b closer view of the overlapped melt pool zones (MPZ); c middle of the MPZ with finer grains; and d closer view of the HAZ and MPZ
4 Conclusions
In this study, L-PBF processing conditions were studied with the aim of optimizing the component density and observing the resultant magnetic properties of NdFeB magnets.
There are defects in the samples, mainly on the sample edges and at the bottom of the samples near the build platform. These defects may result from the temperature differences between the build platform and the hot melted powder above them. The temperature difference between the layers decreases as the build progresses, reducing the thermal stresses on the top of the samples.
A high laser power/high laser scan speed combination of 100 W/3000 mm/s created higher sample density and remanence values than a low laser power/low laser scan speed combination of 60 W/1250 mm/s, both of which resulted in nearly the same energy density. It is concluded that the printing quality is more likely dependent on the laser power; however, increasing the energy density also increases the density of the samples. The greater amount of α -Fe phases detected in the low laser power/low laser scan speed combination can be attributed to the low B r , in addition to the increased lack of fusion pores within them. In addition, it is essential to maintain the laser power above 50 W to melt the powder.
The density of the samples increases with increasing laser power up to 120–130 W and then starts to decrease. It is essential to set a high enough energy density value to melt the powder well. However, it is also essential not to exceed the critical energy density to avoid material boiling and excessive keyhole formation during printing. Therefore, one of the key points in obtaining high-density samples is to maintain the energy density below a critical energy density value. In this study, the critical energy density was 125 J/mm 3 .
Improving the sample density is not the only concern when trying to maximize magnetic performance; there is no linear relationship between the remanence and the density. All the high-magnetism samples had high density, but not all the high-density samples had high magnetism. It can therefore be concluded that the cooling rates, hence the phases and the microstructure, are vital considerations when trying to understand the generated magnetic properties. The energy line ( laser power/laser scan speed ) is another important parameter that needs to be considered. This study revealed that an energy line between 0.03 and 0.04 Ws/mm resulted in a higher remanence. In contrast, the remanence tends to decrease with increasing energy to 0.05 Ws/mm. It was found that the higher B r samples were obtained with energy lines between 0.03 and 0.04 Ws/mm and with energy densities between 75 and 100 J/mm 3 , while the B r values decreased with increasing energy lines to 0.05 Ws/mm and energy densities to 125 J/mm 3 . It is also concluded that the high- B r samples are a result of faster scans.
The magnetic properties are highly dependent on Nd 2 Fe 14 B, which is a strong magnetic phase and a magnetically soft α -Fe phase. A higher magnetic phase (Nd 2 Fe 14 B) was detected in the samples with higher B r and H ci values. In contrast, the lower α -Fe phase found in the samples has higher B r and H ci values.
Up to 95.72% dense samples were obtained with an energy density below 100 J/mm 3 and an energy line between 0.028 and 0.45 Ws/mm.
The maximum properties obtained by parameter optimization are B r 0.72 T and BH max 81 kJ/mm 3 . These are the highest properties obtained in the published literature to date with MQP-S-11–9-20,001 by L-PBF without postprocessing or element doping.
The density percentage of the samples improved with the use of the heated bed (increasing from 90 to 96%). However, no linear relationship is observed within the elevated bed temperature range and density increase. The instability of the heated bed is one of the reasons for fluctuations in the density of the samples.
The remanence of the samples increased with the heated bed, while the intrinsic coercivity decreased. The former is related to the sample density increase and possibly a higher θ phase, and the latter is related to the larger and more dendritic grains in the heated bed samples. B r is related to the θ phase in the samples, while intrinsic coercivity is related to the phase distribution and homogeneity and the composition of the grain boundary phases. The non-uniform and irregular Nd-rich phase reduces the coercivity.
In this paper, while changes in grain morphology and their impact on coercivity were partially elucidated through SEM images of heated bed samples, a comprehensive investigation, including EBSD analyses, would significantly enhance the understanding of the influence of laser parameters on grain morphology [ 58 , 59 ]. A more in-depth analysis, encompassing aspects such as grain size, grain shape, orientation, and the consequential alterations in magnetic properties, is a good recommendation for future studies. Exploring the grain texture of LPBF-produced NdFeB and its correlation with magnetic properties would contribute valuable insights to the evolving field of additive manufacturing of NdFeB magnets.
It was observed that the dendritic-shaped grains are larger and greater in number in the high-temperature-heated bed samples due to their slower cooling rates.
The maximum properties obtained by the heated bed are as follows: B r , 0.76 T; H ci , 750 kA/m; and ( BH) max , 84 kJ/m 3 at 400 °C.
Systematic experiments with variations in bed temperature and relevant printing parameters are needed to determine the optimal temperature range for effective layer adhesion and minimal delamination on top of the samples while achieving the desired magnetic properties.
L-PBF processing of NdFeB powder is highly sensitive and challenging due to the rapid cooling rates generated during this process. The magnetic properties can be improved by optimizing the process parameters and through the use of a heated bed. However, the magnetic properties of these materials are still lower than those of their conventionally sintered counterparts, which are approximately B r 1–1.3 T and H ci 870–2700 kA/m (data taken from Arnold Magnetic Technologies).
Data availability
The data that support the findings of this study are available from the corresponding author, Kübra Genç, upon reasonable request.
Rahman MA (1993) Modern electric motors in electronic world IECON Proceedings (Industrial Electronics Conference) 2:644-648. https://doi.org/10.1109/iecon.1993.339004
Yoshida K, Hita Y, Kesamaru K (2000) Eddy-current loss analysis in PM of surface-mounted-PM SM for electric vehicles. IEEE Trans Magn 36(4 PART 1):1936–1940. https://doi.org/10.1109/20.877826
Article Google Scholar
Hu Y, Zhu S, Liu C (2017) Magnet eddy-current loss analysis of interior PM machines for electric vehicle application. IEEE Trans Magn 53(11):1–4. https://doi.org/10.1109/TMAG.2017.2700850
Wang XWX, Li JLJ, PSP Song (2006) Design optimization and eddy current losses analysis of permanent magnets in PMSM. 2006 12th Biennial IEEE Conference on Electromagnetic Field Computation, vol. 38, no. 2, p. 4244, https://doi.org/10.1109/CEFC-06.2006.1632979
Yin H, Huang S, Li H (2018) Study on the influence of internal water cooling system on the loss of permanent magnet synchronous motor. AIP Conf Proc 1971. https://doi.org/10.1063/1.5041185
Srinivas K, Murtaza Q, Aggarwal AK (2019) Effect of shape of magnet on the machining of workpiece. Int J Recent Technol Eng 8(2 Special Issue 11):2909–2913. https://doi.org/10.35940/ijrte.B1367.0982S1119
Amin S, Madanzadeh S, Khan S, Bukhari SSH, Akhtar F, Ro JS (2022) Effect of the magnet shape on the performance of coreless axial flux permanent magnet synchronous generator. Electr Eng 104(2):959–968. https://doi.org/10.1007/s00202-021-01338-x
Jaćimović J et al (2017) Net shape 3D printed NdFeB permanent magnet. Adv Eng Mater 19(8):1–7. https://doi.org/10.1002/adem.201700098
Brown D, Ma BM, Chen Z (2002) Developments in the processing and properties of NdFeb-type permanent magnets. J Magn Magn Mater 248(3):432–440. https://doi.org/10.1016/S0304-8853(02)00334-7
Access O (2011) The preparation of sintered NdFeB magnet with high-coercivity and high temperature-stability The preparation of sintered NdFeB magnet high-coercivity and high temperature-stability, https://doi.org/10.1088/1742-6596/266/1/012052
Li WF, Ohkubo T, Hono K, Sagawa M (2009) The origin of coercivity decrease in fine grained Nd-Fe-B sintered magnets. J Magn Magn Mater 321(8):1100–1105. https://doi.org/10.1016/j.jmmm.2008.10.032
Oono N, Sagawa M, Kasada R, Matsui H, Kimura A (2011) Production of thick high-performance sintered neodymium magnets by grain boundary diffusion treatment with dysprosium-nickel-aluminum alloy. J Magn Magn Mater 323(3–4):297–300. https://doi.org/10.1016/j.jmmm.2010.09.021
Lee MW, Bae KH, Lee SR, Kim HJ, Jang TS (2017) Microstructure and magnetic properties of NdFeB sintered magnets diffusion-treated with Cu/Al mixed DyCo alloy-powder. Arch Metall Mater 62(2):1263–1266. https://doi.org/10.1515/amm-2017-0189
Paranthaman MP et al (2016) Binder jetting : a novel NdFeB bonded magnet fabrication process. 68(7):1978–1982. https://doi.org/10.1007/s11837-016-1883-4
Brown DN (2016) Fabrication, processing technologies, and new advances for RE-Fe-B magnets. IEEE Trans Magn 52(7):1–9. https://doi.org/10.1109/TMAG.2016.2535482
Huber C et al (2016) 3D print of polymer bonded rare-earth magnets, and 3D magnetic field scanning with an end-user 3D printer. Appl Phys Lett 109(16). https://doi.org/10.1063/1.4964856
Li L et al (2016) Big area additive manufacturing of high performance bonded NdFeB magnets. Sci Rep 6:1–7. https://doi.org/10.1038/srep36212
Li L, Post B, Kunc V, Elliott AM, Paranthaman MP (2017) Additive manufacturing of near-net-shape bonded magnets: prospects and challenges. Scr Mater 135:100–104. https://doi.org/10.1016/j.scriptamat.2016.12.035
Bittner F, Thielsch J, Drossel WG (2020) Laser powder bed fusion of Nd–Fe–B permanent magnets. Prog Addit Manuf 5(1):3–9. https://doi.org/10.1007/s40964-020-00117-7
Urban N, Meyer A, Kreitlein S, Leicht F, Franke J (2017) Efficient near net-shape production of high energy rare earth magnets by laser beam melting. Appl Mech Mater 871:137–144. https://doi.org/10.4028/www.scientific.net/amm.871.137
Skalon M et al (2019) Influence of melt-pool stability in 3D printing of NdFeB magnets on density and magnetic properties. Materials 13(1):139. https://doi.org/10.3390/ma13010139
Jacimovic J, Christen T, Dénervaud E (2020) Self-organized giant magnetic structures via additive manufacturing in NdFeB permanent magnets. Addit Manuf 34(May):101288. https://doi.org/10.1016/j.addma.2020.101288
Popov V, Koptyug A, Radulov I, Maccari F, Muller G (2018) Prospects of additive manufacturing of rare-earth and non-rare-earth permanent magnets. Procedia Manuf 21(2017):100–108. https://doi.org/10.1016/j.promfg.2018.02.199
Cordero ZC, Meyer HM, Nandwana P, Dehoff RR (2017) Powder bed charging during electron-beam additive manufacturing. Acta Mater 124:437–445. https://doi.org/10.1016/j.actamat.2016.11.012
Zhao X, Dadbakhsh S, Rashid A (2021) Contouring strategies to improve the tensile properties and quality of EBM printed Inconel 625 parts. J Manuf Process 62:418–429. https://doi.org/10.1016/j.jmapro.2020.12.007
Radulov IA et al (2019) Production of net-shape Mn-Al permanent magnets by electron beam melting. Addit Manuf 30:100787. https://doi.org/10.1016/j.addma.2019.100787
Jonner CJ et al (2019) High-quality, high-speed EBM 3D printing by the integration of high-performance electron sources. EBMPerform, no. 666788, pp 1–18. https://ec.europa.eu/research/participants/documents/downloadPublic?documentIds=080166e5cab4848b&appId=PPGMS
Kolb T et al (2016) Laser Beam Melting of NdFeB for the production of rare-earth magnets,” 2016 6th International Electric Drives Production Conference, EDPC 2016 - Proceedings, pp. 34–40. https://doi.org/10.1109/EDPC.2016.7851311
Jaćimović J et al (2017) Net shape 3D printed NdFeB permanent magnet. Adv Eng Mater 19(8). https://doi.org/10.1002/adem.201700098
Tang XT, Lu ZW, Sun AZ (2019) The effect of sintered Nd-Fe-B with Dy infiltration to the plating crafts. J Magn Magn Mater 475:10–13. https://doi.org/10.1016/j.jmmm.2018.11.080
Cui XG et al (2017) Magnetic properties and microstructure of sintered Nd–Fe–B magnets with intergranular addition of Ni powders. J Alloys Compd 726:846–851. https://doi.org/10.1016/j.jallcom.2017.08.061
Zhang P, Liang L, Jin J, Zhang Y, Liu X, Yan M (2014) Magnetic properties and corrosion resistance of Nd-Fe-B magnets with Nd64Co36intergranular addition. J Alloys Compd 616:345–349. https://doi.org/10.1016/j.jallcom.2014.07.085
Jin C et al (2016) Magnetic properties and phase evolution of sintered Nd-Fe-B magnets with intergranular addition of Pr–Co alloy. J Alloys Compd 670:72–77. https://doi.org/10.1016/j.jallcom.2016.02.006
Zhang P, Ma T, Liang L, Yan M (2014) Influence of Ta intergranular addition on microstructure and corrosion resistance of Nd-Dy-Fe-B sintered magnets. J Alloys Compd 593:137–140. https://doi.org/10.1016/j.jallcom.2014.01.055
Volegov AS et al (2020) Additive manufacturing of heavy rare earth free high-coercivity permanent magnets. Acta Mater 188:733–739. https://doi.org/10.1016/j.actamat.2020.02.058
Vial F, Joly F, Nevalainen E, Sagawa M, Hiraga K, Park KT (2002) Improvement of coercivity of sintered NdFeB permanent magnets by heat treatment. J Magn Magn Mater 242–245:1329–1334. https://doi.org/10.1016/S0304-8853(01)00967-2
Zhang Y et al (2018) Post-sinter annealing influences on coercivity of multi-main-phase Nd-Ce-Fe-B magnets. Acta Mater 146:97–105. https://doi.org/10.1016/j.actamat.2017.12.027
Goll D, Trauter F, Bernthaler T, Schanz J, Riegel H, Schneider G (2021) Additive manufacturing of bulk nanocrystalline FeNdB based permanent magnets. Micromachines (Basel) 12(5). https://doi.org/10.3390/mi12050538
Tosoni O et al (2023) High-coercivity copper-rich Nd-Fe-B magnets by powder bed fusion using laser beam method. Addit Manuf 64. https://doi.org/10.1016/j.addma.2023.103426
Pelevin IA et al (2023) New scanning strategy approach for laser powder bed fusion of Nd-Fe-B hard magnetic material. Metals (Basel) 13(6). https://doi.org/10.3390/met13061084
Yu KS et al (2022) Additive manufacturing of NdFeB magnets by synchronized three-beam laser powder bed fusion. Opt Laser Technol 146. https://doi.org/10.1016/j.optlastec.2021.107604
Fraser CJ (2002) Electrical machines. The Mechatronics Handbook, pp. 20–33–20–51. https://doi.org/10.1201/9781420037043-13
Pereira T, Kennedy JV, Potgieter J (2019) A comparison of traditional manufacturing vs additive manufacturing, the best method for the job,” in Procedia Manufacturing, Elsevier B.V., pp. 11–18. https://doi.org/10.1016/j.promfg.2019.02.003
Zhou X, Paranthaman MP, Sutherland JW (2023) Comparative techno-economic assessment of NdFeB bonded magnet production: injection molding versus big-area additive manufacturing. ACS Sustain Chem Eng 11(36):13274–13281. https://doi.org/10.1021/acssuschemeng.3c01942
Rong C, Shen B (2018) Nanocrystalline and nanocomposite permanent magnets by melt spinning technique. Chin Phys B 27(11):117502. https://doi.org/10.1088/1674-1056/27/11/117502
Urban N, Huber F, Franke J (2017) Influences of process parameters on rare earth magnets produced by laser beam melting. 2017 7th International Electric Drives Production Conference, EDPC 2017 - Proceedings, vol. 2017-Decem, pp. 1–5, 2018, https://doi.org/10.1109/EDPC.2017.8328149
Wu J, Aboulkhair NT, Degano M, Ashcroft I, Hague RJM (2021) Process-structure-property relationships in laser powder bed fusion of permanent magnetic Nd-Fe-B. Mater Des 209:109992. https://doi.org/10.1016/j.matdes.2021.109992
Urban N, Meyer A, Keller V, Franke J (2018) Contribution of additive manufacturing of rare earth material to the increase in performance and resource efficiency of permanent magnets. Appl Mech Mater 882:135–141. https://doi.org/10.4028/www.scientific.net/amm.882.135
Bittner F, Thielsch J, Drossel WG (2021) Microstructure and magnetic properties of Nd-Fe-B permanent magnets produced by laser powder bed fusion. Scr Mater 201:113921. https://doi.org/10.1016/j.scriptamat.2021.113921
Waqar S, Guo K, Sun J (2022) Evolution of residual stress behavior in selective laser melting (SLM) of 316L stainless steel through preheating and in-situ re-scanning techniques. Opt Laser Technol 149:107806. https://doi.org/10.1016/j.optlastec.2021.107806
Ali H, Ma L, Ghadbeigi H, Mumtaz K (2017) In-situ residual stress reduction, martensitic decomposition and mechanical properties enhancement through high temperature powder bed pre-heating of selective laser melted Ti6Al4V. Mater Sci Eng, A 695(April):211–220. https://doi.org/10.1016/j.msea.2017.04.033
Yue H (2022) Effects of energy density on the mechanical properties, residual stress and thermal-fatigue of Fe-Cr alloy fabricated by laser directed energy deposition, pp 0–31. https://doi.org/10.21203/rs.3.rs-2342095/v1
Zhang S, Xu S, Zheng W, Han J (2022) Study of the effect of SLM energy density on residual stress and microstructure of porous bone scaffolds in cubic structures. Int J Simulat Multidiscipl Des Optim 13. https://doi.org/10.1051/smdo/2022016
Chen C, Chang S, Zhu J, Xiao Z, Zhu H, Zeng X (2020) Residual stress of typical parts in laser powder bed fusion. J Manuf Process 59:621–628. https://doi.org/10.1016/j.jmapro.2020.10.009
Oster NT (2012) Generation and characterization of anisotropic microstructures in rare earth-iron-boron alloys, p 134. https://doi.org/10.31274/etd-180810-2741
Lewis LH, Jiménez-Villacorta F (2013) Perspectives on permanent magnetic materials for energy conversion and power generation. Metall Mater Trans A Phys Metall Mater Sci 44(SUPPL. 1). https://doi.org/10.1007/s11661-012-1278-2
Fidler J, Skalicky P (1987) Metallurgical factors determining the coercivity of ND-FE-B magnets. Mikrochim Acta 91:115–124. https://doi.org/10.1007/BF01199483
Pérez-Ruiz J, González-Barrio H, Sanz-Calle M, Gómez-Escudero G, Munoa J, de Lacalle LL (2023) Machining stability improvement in LPBF printed components through stiffening by crystallographic texture control. CIRP Ann 72(1):141–144. https://doi.org/10.1016/j.cirp.2023.03.025
Pérez-Ruiz JD, de Lacalle LNL, Urbikain G, Pereira O, Martínez S, Bris J (2021) On the relationship between cutting forces and anisotropy features in the milling of LPBF Inconel 718 for near net shape parts. Int J Mach Tools Manuf 170. https://doi.org/10.1016/j.ijmachtools.2021.103801
Download references
Acknowledgements
The authors gratefully acknowledge Justin Mitchell and Doug Walston from Arnold Magnetic Technologies for Helmholtz coil tests /Magnetic Tests. The authors would like to thanks the EPSRC Future Manufacturing Hub in Manufacture using Advanced Powder Processes (MAPP) (EP/P006566/1) for their support during this investigation.
This study was funded by the Republic of Türkiye Ministry of National Education, HiETA Technologies Limited, and Equipmake.
Author information
Authors and affiliations.
Departmant of Mechanical Engineering, University of Sheffield, Sheffield, S1 4BJ, UK
Kübra Genç & Kamran Mumtaz
Department of Materials Science and Engineering, University of Sheffield, Sheffield, S1 4BJ, UK
Department of Materials Science and Metallurgy, University of Cambridge, Cambridge, CB3 0FS, UK
Sirapob Toyting
Department of Mechanical Engineering, University College London, London, WC1E 7JE, UK
Enrique Galindo-Nava
You can also search for this author in PubMed Google Scholar
Contributions
Conceptualization, Kübra Genç; data curation, Kübra Genç and Sirapob Toyting; formal analysis, Kübra Genç; funding acquisition, Kamran Mumtaz; investigation, Kübra Genç and Sirapob Toyting; methodology, Kübra Genç; project administration, Kübra Genç; supervision, Kamran Mumtaz and Iain Todd; validation, Kübra Genç; visualization, Kübra Genç and Sirapob Toyting; writing—original draft, Kübra Genç; and writing—review and editing, Kamran Mumtaz and Enrique Galindo-Nava.
Corresponding author
Correspondence to Kübra Genç .
Ethics declarations
Competing interests.
The authors declare no competing interests.
Additional information
Publisher's note.
Springer Nature remains neutral with regard to jurisdictional claims in published maps and institutional affiliations.
Sets of parameters based on the studies by Urban et al. and Kolb et al. Process parameters not numbered in the table were excluded from the initial stage of the experiment since their respective EL values fell outside the range of 0.03–0.05 Ws/mm
Rights and permissions.
Open Access This article is licensed under a Creative Commons Attribution 4.0 International License, which permits use, sharing, adaptation, distribution and reproduction in any medium or format, as long as you give appropriate credit to the original author(s) and the source, provide a link to the Creative Commons licence, and indicate if changes were made. The images or other third party material in this article are included in the article's Creative Commons licence, unless indicated otherwise in a credit line to the material. If material is not included in the article's Creative Commons licence and your intended use is not permitted by statutory regulation or exceeds the permitted use, you will need to obtain permission directly from the copyright holder. To view a copy of this licence, visit http://creativecommons.org/licenses/by/4.0/ .
Reprints and permissions
About this article
Genç, K., Toyting, S., Galindo-Nava, E. et al. Laser powder bed fusion of NdFeB and influence of powder bed heating on density and magnetic properties. Int J Adv Manuf Technol (2024). https://doi.org/10.1007/s00170-024-13605-9
Download citation
Received : 01 January 2024
Accepted : 06 April 2024
Published : 29 April 2024
DOI : https://doi.org/10.1007/s00170-024-13605-9
Share this article
Anyone you share the following link with will be able to read this content:
Sorry, a shareable link is not currently available for this article.
Provided by the Springer Nature SharedIt content-sharing initiative
- Additive manufacturing
- NdFeB magnets
- Laser powder bed fusion (L-PBF)
- Find a journal
- Publish with us
- Track your research

IMAGES
VIDEO
COMMENTS
The paper becomes magnetized because the elastic rubber or plastic on the back is embedded with magnetized strontium ferrite powder, or some other magnetized material. Basically, millions of tiny magnets create a flexible magnetic sheet! The thickness of the magnet designates its strength, but thicker types of magnet paper are more limited in ...
Bar Magnet and Magnetic Field Lines: The direction of magnetic field lines represented by the alignment of iron filings sprinkled on paper placed above a bar magnet. Various phenomena have the effect of "displaying" magnetic field lines as though the field lines are physical phenomena.
If a magnetic object like a paper clip enters a magnetic field, it is pulled toward the magnet. Magnetic fields even work under water. A strong magnet can even attract a magnetic object through a table. Advertisement. Magnets Have Two Poles: North and South. A magnet has two ends, called poles. One end is the north pole, and the other is the ...
Magnetic paper is a fascinating innovation that combines the properties of magnets with the flexibility and usability of paper. It has a myriad of applications, ranging from educational tools to creative projects and functional items. In this article, we will delve into the inner workings of magnetic paper and explore its various uses. ...
Magnetic lines of force of a bar magnet shown by iron filings on paper Detecting magnetic field with compass and with iron filings. The phenomenon of magnetism is "mediated" by the magnetic field. An electric current or magnetic dipole creates a magnetic field, and that field, in turn, imparts magnetic forces on other particles that are in the ...
The bar magnet and paper clips from Figure 9 are examples of permanent and temporary magnets, respectively. The bar magnet is always surrounded by a magnetic field, so it is a permanent magnet. The paper clips do not normally have a magnetic field; in other words, you cannot use one paper clip to pick up another paper clip.
The side of the magnet from which the field lines emerge is defined as the north pole.The main advantage of an electromagnet over a permanent magnet is that the magnetic field can be rapidly manipulated over a wide range by controlling the amount of electric current; a continuous supply of electrical energy is required to maintain the field.
Key Takeaways: How Magnets Work. Magnetism is a physical phenomenon by which a substance is attracted or repelled by a magnetic field. The two sources of magnetism are electric current and spin magnetic moments of elementary particles (primarily electrons). A strong magnetic field is produced when the electron magnetic moments of a material are ...
Describe how magnetic poles interact with each other. Figure 22.1.1 22.1. 1: Magnets come in various shapes, sizes, and strengths. All have both a north pole and a south pole. There is never an isolated pole (a monopole). All magnets attract iron, such as that in a refrigerator door. However, magnets may attract or repel other magnets.
Magnetic materials near a magnet also act as it they were magnets. For instance, a paper clip hanging from a magnet attracts other paper clips as illustrated on the right. The first paper clip is an example of a temporary magnet. Figure 38.2.1.
That is what is happening in our experiment with the iron filings. Each tiny magnetic iron filing is a tiny magnet with a north and south pole, just like a tiny compass. When the iron filings are sprinkled, those very close to the magnet, where the magnetic force is the strongest, will cling to the magnet. Those filings a little farther away ...
A magnet is a material or object that produces a magnetic field.This magnetic field is invisible but is responsible for the most notable property of a magnet: a force that pulls on other ferromagnetic materials, such as iron, steel, nickel, cobalt, etc. and attracts or repels other magnets.. A permanent magnet is an object made from a material that is magnetized and creates its own persistent ...
However, when the magnet is removed, the paper clips will no longer act as magnets. Magnetic Fields. Every magnet creates an invisible magnetic field around it. The area around the magnet that exerts magnetic force is known as the magnetic field. Suppose you put a bar magnet on a table and put a paper clip nearby.
Magnetic paper is a versatile and innovative material that combines the convenience of a printable surface with the functionality of a magnet. Whether you're a creative enthusiast, a teacher, or a professional looking for unique solutions, magnetic paper can revolutionize the way you approach various projects. In this article, we will provide ...
A magnetic pole is the part of a magnet that exerts the strongest force on other magnets or magnetic material, such as iron. For example, the poles of the bar magnet shown in Figure 20.2 are where the paper clips are concentrated.
Now, rub a paper clip with the magnet. Can you pick up other paper clips with it now? The iron in the paper clip can become a magnet too: the paper clip is magnetized by rubbing it with the magnet. It doesn't magnetize all at once though: when you rub it longer, it gets more magnetized and picks up other paper clips better and better. Magnetic ...
This force, known as magnetism, is what draws the magnet to certain materials, including the paper clip. The magnet's ability to attract and repel objects is a fundamental aspect of its nature. The magnet's magnetic field is created by the alignment of its atoms, which have tiny magnetic fields of their own.
Placing the magnets under a piece of paper and sprinkling iron filings on top, we will visualize the magnetic field's lines and observe the distinctive patterns formed by the iron filings. In Part 3, we will investigate how the magnetic force influences the displacement of iron filings along both the x-axis and y-axis. By placing the iron ...
The magnet is permanent, and the paper clip is not. However, the magnetic field from the magnet can temporarily magnetize the paper clip. For a short time afterward, the paper clip has a magnetic charge that enables it to attract other paper clips. Electromagnetism is essential in creating specific temporary magnets. The strong magnets often at ...
100 °C (the temperature of boiling water) You will use the amount of paper clips that the magnet can pick up as a measure of its strength. Because your magnet might pick up quite a few paper clips, you will not count the number of clips, but will use their total mass, expressed in grams (g) as your unit of measurement.
Use a paper towel holder to hold the magnet wire spool while winding your magnets, or make an improvised one using a pencil and a cardboard box (Figure 3). This will help you keep your coils neat and prevent knots in the wire. Leave a tail of wire—at least 6 cm long—at each end of the coil. You will use these wire tails to connect the coil ...
Once the paper clip is released, the magnet regains the potential energy it lost. In summary, the potential energy gained by the paper clip when it jumps up to the magnet comes from the energy density of the magnetic field, which is slightly reduced by the paper clip. When the paper clip is pulled off the magnet, the magnet regains this ...
Inkjet magnetic paper is a specially designed material that combines the convenience of printable paper with the magnetic properties of magnets. It allows you to print your designs, artwork, or text using an inkjet printer and then turn them into magnets or other magnetic materials. The printable side of the paper is coated to receive ink from ...
A lot has changed at Fort Worth's Colonial Country Club since it hosted the 2023 Charles Schwab Challenge. The storied course that Marvin Leonard opened in 1936, and then introduced in legendary ...
View a PDF of the paper titled Simulating Spin Dynamics of Supersolid States in a Quantum Ising Magnet, by Yi Xu and 3 other authors. ... We map out the ground state phase diagram and compute the dynamical spin structure factors across a range of magnetic field strengths, focusing especially on the two supersolid phases found near zero and ...
Please use one of the following formats to cite this article in your essay, paper or report: APA. Sepmag. (2024, May 09). Unlocking efficiency: The imperative of scaling up magnetic beat ...
An unusual reduction in the strength of Earth's magnetic field between 591 and 565 million years ago coincided with a significant increase in the oxygen levels in the atmosphere and oceans ...
Laser powder bed fusion (L-PBF) is an additive manufacturing technique that provides an opportunity to create complex NdFeB magnets, potentially enhancing their performance. L-PBF possesses its own processing challenges, such as porosity/cracks and thermal stresses due to rapid cooling. This study focused on optimizing the parameters and the use of elevated temperature (300-550 °C) powder ...